In the early eighteenth century the most noteworthy connection between electricity and nervous function was that very little was known about either. Based on the ideas of the time, there was little reason to suspect a deep relationship between the two. Electricity required the possibility of a spark. The brain operated via a mechanism of fluid movement and pressure [1]. There was, however, one puzzling observation.
It had been shown many times that, under experimental conditions, an electric spark could elicit muscular contraction [2]. Naturally, this led some to consider whether a relationship existed between the two, and in doing so, a new scientific field was born.
Luigi Galvani was not the first electrophysiologist. However, when he took up the problem around 1780, he became one of the most important early pioneers. By devising a clever experiment, in which he exposed the nerves of a frog’s lower limbs, Galvani was able to show that man-made and natural (e.g. lighting) electricity led to muscular contraction [2].
The Discovery
Fifty years passed between Galvani’s initial experiments and the next substantial electrophysiological advancement; the discovery of the action potential. Following
up on the work of Carlo Matteucci, which had convincingly supported Galvani’s ideas, Emil du Bois-Reymond was able to make the final connection between electricity and nervous function [2].
Bois-Reymond, a skilled experimentalist with access to high-quality instruments, was able to demonstrate that, similar to muscle tissue, nerve fiber current decreased when stimulated. Though he could not demonstrate the time-course of the depolarization (Figure 3) with sufficient precision, Bois-Reymond correctly hypothesized that rapid reversal in cell polarity might travel down the nerve fiber. He claimed, “If I do not greatly deceive myself, I have succeeded in realizing... the hundred years’ dream of physicists and physiologists, to wit, the identity of the nervous principle with electricity” [2].
The Squid Giant Axon
Of course since Bois-Reymond many brilliant researchers have further illuminated our understanding of the action potential, its ion channels, and lifespan. Alan Hodgekin and Andrew Huxley, who shared the Nobel Prize for their work, famously studied the squid giant axon to expose the detailed ionic mechanisms of the action potential. As can be easily seen in figure 1, the squid is equipped with an axon 1,000 times wider than is typical (up to 1mm vs. 1μm). The evolutionary benefit of such an enlarged axon is the increased speed, which the squid uses to activate its jet propulsion system and escape danger. (For a brief explanation of the physics behind the velocity-diameter relationship, see the figure 1 caption.)
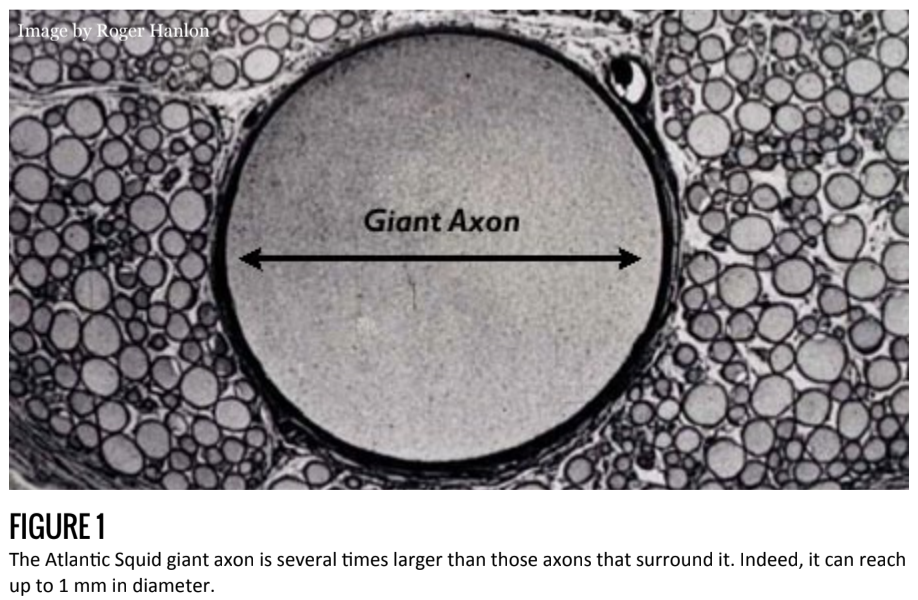
The scientific benefit of such a research model, however, is the experimental advantage conferred by the large diameter. Measuring voltage and current within the axon is a technically difficult task that requires inserting electrodes into the lumen of the axon. Obviously, this is more easily accomplished in the giant axon.
Today the contributions of Hodgekin and Huxley remain one of the major advances in neuroscience. For, as will be discussed below, the action potential is the currency of information conductance in the nervous system. Their work set the stage, allowing it to be a well-understood biophysical phenomenon.
The Structural Elements
Before discussing the action potential proper, it is important to first become familiarized with the structural components that permit its existence: the plasma membrane, ion channels, and pumps.
While it might be easy to overlook the plasma membrane, it plays a critical role by maintaining the necessary inside-to-outside charge imbalance. As a lipid bilayer, the membrane is impermeable to charged ions such as sodium (Na+), potassium (K+), and chloride (Cl-). Without such a barrier, ions would flow freely between the cell and its environment, dissipating any voltage between the two.
Ion channels are trans-membrane proteins that act as conduits through which specific ions may flow. While there are hundreds of known human channels [3], their structure and function are broadly the same. Each channel is capable of an open or closed configuration, which acts as a gate. Only when it is open are ions – specific to that channel – able to flow, in either direction, through it. When closed, the membrane is again impermeable to that ion.
The wide variation in ion channels is largely due to the specificity of stimuli that can open or close gates. Voltage, current, mechanical force, concentration gradients, and pH are all examples of stimuli that can modulate the configuration of a channel. For example, touch is a sensation whose signaling begins with the application of mechanical force, which opens channels to ion flow. In order to reestablish the resting electric potential across the membrane, nerves rely on pumps to actively transport ions into and out of the cell. Such movement is typically against the energy gradient and requires metabolic energy (ATP) to complete. One such example is the well-known sodium-potassium
pump.
The Action Potential
The action potential is a short-lasting event in which the typically negative (relative to outside the cell) membrane potential rapidly rises, becomes positive, and falls again. It is the method of information conveyance over long distances. Some axons can be more than one meter long.
There are four properties that guide the initiation and propagation of action potentials. First, there is a threshold of initiation. Second, it is an all-or-nothing event. Third, it has constant amplitude. Fourth, every action potential is followed by a brief refractory period [4].
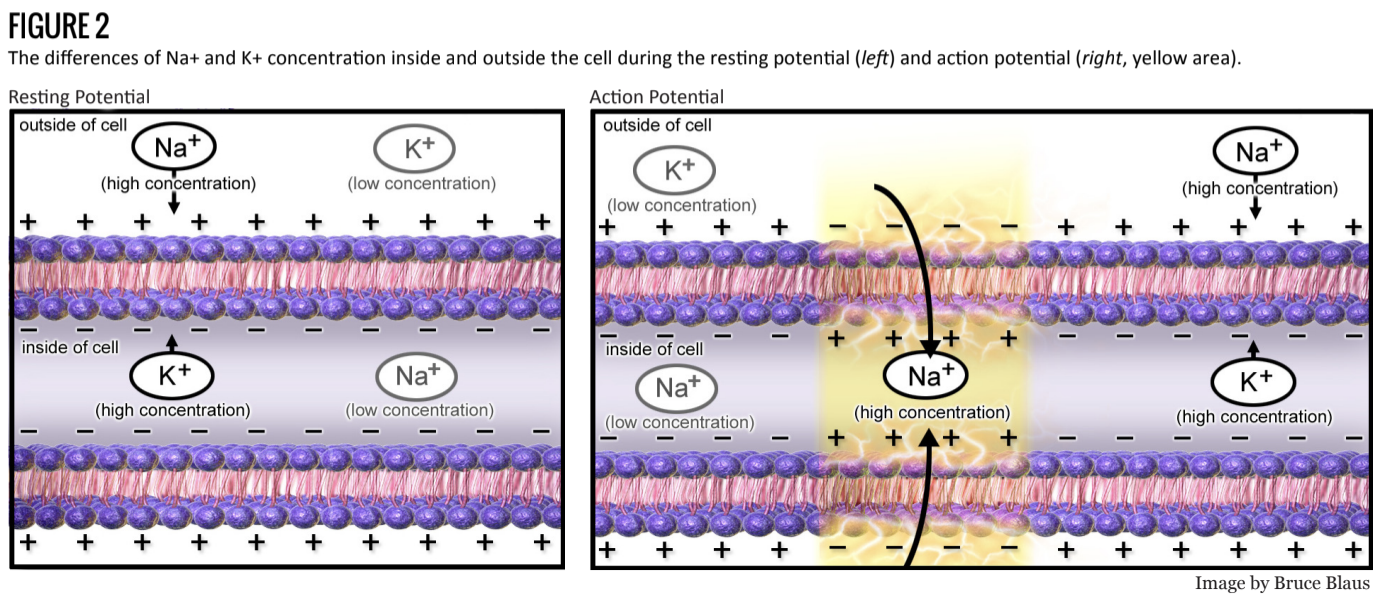
The behavior of the action potential depends several properties, including the electrical potential and chemical concentration of several ions, and the configuration of ion channels. As is shown in figure 2, the resting membrane potential is roughly -70mV and due to the Na+/K+ pump. Na+ concentrations are lower within the cell, while K+ is greater.
Each neuron receives excitatory and inhibitory signals. This sum of such signals results in a membrane potential that is either more positive (depolarizes) or negative (hyperpolarizes). This signal competition is an important type of neural processing [4].
Once a neuron receives sufficient excitatory stimulus to reach the neuron’s threshold (around -50mV) voltage-gated Na+ channels open rapidly and the action potential begins.
As Na+ enters the cell, the influx of positive charge depolarizes the cell further, causing additional Na+ channels to open. At the peak voltage (Figure 3.3) Na+ channels close and the voltage-gated K+ channels open. This allows K+, following its electrochemical gradient, to exit the cell [4].
The repolarization phase (Figure 3.4) is characterized by the efflux of K+, and, because at the positive membrane potential voltage-gated Cl- channels open, Cl- ions enter the cell.
This allows the cell to more rapidly return to its base membrane potential as well as introduce a refractory period in which the cell is hyperpolarized (Figure 3.5). This period serves to drive forward propagation as well as limits the frequency of action potentials (another type of neural processing). After the termination of an action potential, the chemical equilibrium is reestablished via pumps such as the Na+/K+ - ATPase (see Figure 2). Downstream, the effect of the action potential leads to exocytosis of neurotransmitter at the synapse. Depending on the excitatory and inhibitory properties of the post-synaptic cell, the action potential continues on.
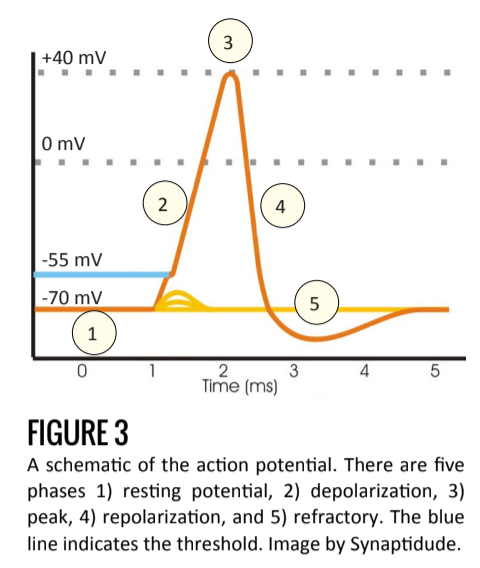
Conclusion
In order to fully understand neurons, neural circuits, and entire nervous systems, it is essential to have a firm grasp on the primary mechanism of their function. This article provides an exceptionally brief overview of the historical discovery and cellular mechanism of the action potential. More detailed information can be found in Eric R. Kandel’s Principles of Neural Science.