Early work in developmental neuroscience led researchers to conclude that axonal growth in the developing and regenerating brain is a specific, targeted process, and not simply random or determined by neuronal proximity. Roger Sperry demonstrated this in 1963 when he cut the optic trunk of a Xenopus frog and rotated the eye 180 degrees [1].
The purpose of this experiment was to understand whether an intrinsic mechanism (as opposed to an external mechanism) is responsible for axonal growth.
If an external stimulus, for example, light, were involved in the regrowth of the circuit, the frog’s field of vision would reorient itself in the correct direction. In time, the frog would see normally despite an inverted retina. But if an internal mechanism were responsible for axonal regeneration, the newly formed connections would not depend on the orientation of the retina and the frog would have an inverted field of vision (Figure 1).
Sperry hypothesized the existence of an exclusive “lock and key” mechanism, where every axon is identified by a unique cellular marker compatible with specific receptors of a target cell. Under such a hypothesis, optic nerve wiring would not change, leading to post-experimental circuitry identical to the pre-experimental connections.
Indeed, Sperry found that after severing and reconnecting the optic nerve, axonal regeneration led to pre-experimental connections, resulting in an inverted field of vision for the frog. He concluded from these results that a molecular or electrical mechanism was at work — guiding axons to target regions and target cells before a synapse is ever formed.
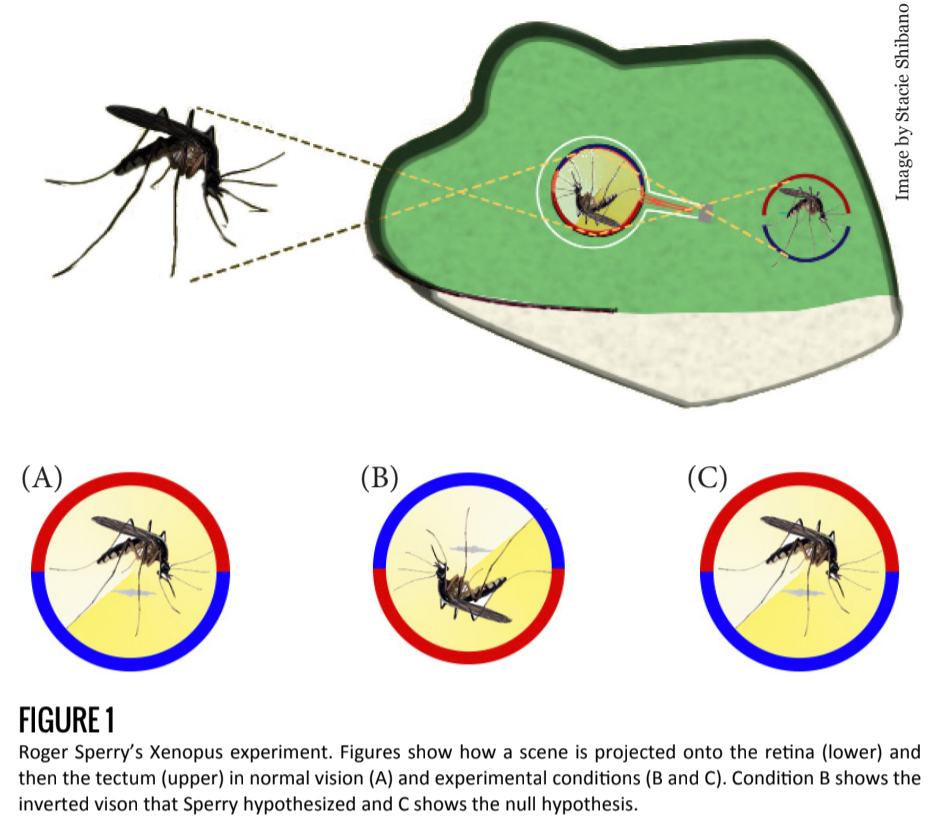
Background and Current Research
This work in the early 1960s paved the way for current understanding of the complex mechanisms of developmental circuitry. Axonal regeneration of the optic nerve is only one example of targeted growth and development in the brain. Sperry’s work showed that neuronal regeneration follows previously made connections, but did not explain how those connections are first established during development.
Over a century ago, Santiago Ramón Y Cajal proposed that developing axons were directed along a particular trajectory. His idea was based on observations of the shape of the growing tip, or growth cone (Figure 2).
Today, we know that when axons grow, the position of its tip – the growth cone – changes, and is in fact responsible for the direction of growth and movement [2]. The growth cone contains a dynamic network of microtubules and actin filaments that mediate the orientation of the growth cone. Actin filaments function through a polymerization and depolymerization mechanism in response to extracellular stimuli via second messengers (molecules or ions, such as calcium ions, that are released within the neuron in response to extracellular ligand-receptor binding.).
Further evidence supporting the role of growth cones in axonal path-finding follows from observations of pioneer neurons, the first set of axons to migrate, and follower neurons, the later sets. In contrast to the large, flattened, and active growth cones of pioneer axons, follower axonal growth cones are much simpler and less extensive. Their shape seems to be pointed in a particular direction. This suggests that pioneering axons behave as trailblazers, laying the path for cells to follow, while follower axons are guided along via cell-to-cell signals.
Follower axons have relatively inactive path-finding mechanisms, indicating that growth is largely undetermined by external stimuli [2]. Furthermore, recent research shows that protein movement within growth cones occurs at a higher rate among following axons than pioneering axons, indicating that axons grow and synapse at a faster rate in the presence of a guidance axon [3] (Figure 3).
When growth cones are actively searching for a target, or trailblazing, growth-signaling molecules have two main effects on axonal growth: repulsion or attraction [2]. Depending on the second messenger pathways that exist in the receptor cell, molecular signals can be diffusible (acting at a distance) or require cell-to-cell contact.
Netrin, for example, is an important signaling molecule in spinal cord development and is a diffusible substance that can act as a repellent or attractant. In the presence of Netrin a growth cone expressing only DCC receptor will turn towards the source of Netrin. If, however, a growth cone co-expresses Unc5 receptor, it will grow in an adjusted direction, usually away from the Netrin [4]. In the developing spinal cord, cells in the floor plate of the ventral midline secrete Netrin, and growing axons respond in a variety of ways.
In contrast, non-diffusible substances, such as Ephrin, require that that growth cones come in nearly direct contact with another neuron to cause an effect. During development of the visual system, for example, retinal axons containing Ephrin receptors bind to membrane-bound Eph ligands in the tectum, usually triggering a repellent response [5].
If axonal regeneration and growth primarily follow a pattern determined during development, there must be intricate cell-to-cell signaling that takes place as a precursor to synaptogenesis. Sperry hypothesized that a unique signal-receptor pair exists for every axon-dendrite synapse pair. However, this is not plausible due to the sheer number of unique molecules that would have to exist for every synapse in a mammalian brain to be uniquely recognized.
Evidence suggests instead that the developing brain is regionalized before neuronal development. Thus, axonal projections from specific regions follow a similar set of patterns and cues throughout their growth [6]. Research in the mid-1990’s showed that differences in gene expression occurs in well-defined areas in the zebrafish forebrain. This differential gene expression leads to predicted neural differentiation projecting from that region [7]. But even in cases of identical gene expression, not all regionally similar axons follow the exact same patterns of growth and migration; there is some spatial variation. For example, axons can migrate toward a specific direction without originating from the exact same area. This suggests that a seemingly homogeneous population of neurons acquire a spatial awareness and behave accordingly. The most recent research suggests that a gradient mechanism is responsible for the majority of axonal growth and recognition mechanisms.
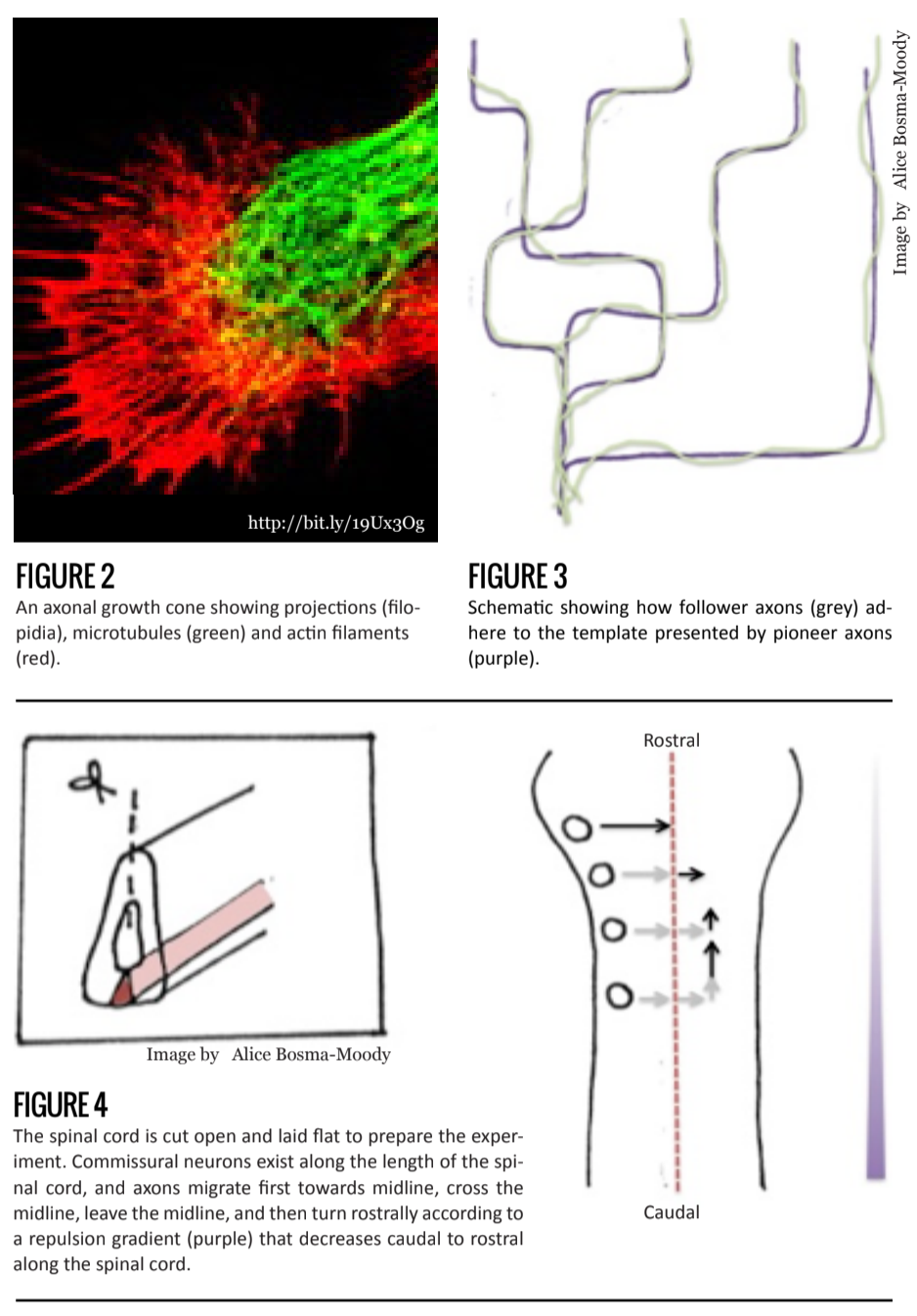
Retinal Organization and Commissural Neuron Growth in the Developing Spinal Cord
Two well-studied areas of development best illustrate the gradient mechanism: visual and spinal development. Before Sperry, scientists had established that axonal projections from the retina to the tectum (or superior colliculus in mammals) are “flipped.” That is, dorsal retinal axons project to the ventral tectum and ventral retinal axons project to the dorsal tectum. Similarly, anterior retinal axons and the posterior tectum are connected, as are posterior retinal axons and the anterior tectum.
A group examined the nature of ligand-receptor interactions among tectal and retinal axons and found it to be repulsive. It was observed that posterior retinal axons contain high levels of Eph receptor, while anterior retinal axons contain little to no Eph receptor. Furthermore, the absolute amount of Eph receptor expressed was found to decrease posterior to anterior in the retina.
The expression of Ephrin ligand, which binds to Eph receptors, was found to be highest in the posterior tectum, and almost non-existent in the anterior tectum. These observations, coupled with the repulsion hypothesis, led researchers to conclude that ligand-expressing anterior cells repel posterior retinal axons. To explain why anterior retinal axons — which display no preference for tectal placement — group at the posterior tectum, researchers hypothesized that competition from posterior retinal axons forced anterior retinal axons to the posterior [8].
Another example of a gradient mechanism that orders a large, homogeneous population of neurons comes from studies of commissural neurons in the developing spinal cord. These neurons function in sensory integration from the spinal cord to the brain and are located in bundles on one side of the developing spinal cord.
Commissural axons migrate along a specific path. Their axons extend toward the ventral midline, traverse through that midline to the contralateral side, then immediately turn 90 degrees and extend rostrally. It was found that two separate signaling molecules were responsible first, for growth towards the midline and then, away from the it. The rostral extension, however, puzzled scientists—all commissural axons behaved in this way, but these bundles were distributed throughout the length of the spinal cord. If one signaling molecule were responsible for this, as a common response would suggest, there is no way to determine in which way, rostral or c, the commissural axons would turn.
Once again, a gradient mechanism is in fact responsible. Wnt protein decreases in concentration along the midline caudal to rostral, and repels commissural axons. Axonal growth thus occurs in the direction of decreasing Wnt concentration. This allows every commissural bundle, irrespective of rostro-caudal position to migrate rostrally [9] (Figure 4).
Conclusion
The result is general. Axonal growth, targeting, and differentiation is dependent upon two important factors: spatial organization, due to the expression of a unique population of genes in each region, and signal gradients. Such gradient mechanisms in signaling are emerging in current research as a common theme of axonal growth, and development in general. The original “lock and key” mechanism hypothesized by Roger Sperry nearly fifty years ago is continually revised by new observations in the mechanisms of recognition, growth, and targeting.
The developing brain is remarkably consistent among individuals and even among different species. Axonal recognition and targeted synaptogenesis in the early stages of development are essential to the creation of the basic and fixed neural circuits that are consistent between individuals. Variations based on experience then builds on those fixed circuits and refines those basic connections.