What makes cows go mad? The deceptively short answer: prions. Most of the human population has encountered pathogenic viruses and bacteria at some point in their lives, but very few have experienced an illness related to prions, which are misfolded proteins. That is a relief, since no prion disease will pass in the same way as the common cold. Prion diseases, such as mad cow disease, are rare, but fatal.
The name mad cow disease, also known as bovine spongiform encephalopathy (BSE), hints at the features of the illness. The affected patients experience neurodegeneration, the loss of structure and function in neurons. While most people probably do not eat cow brains (although who knows what could be in that burger), consumption of BSE infected meat can cause an illness called variant Creutzfeldt-Jakob Disease in humans. The neurological symptoms for this disease include dementia and poor coordination, resulting in death [1]. Unlike other transmissible illnesses, prion diseases occur sporadically and can be genetically passed down. Collectively, these prion diseases are known as transmissible spongiform encephalopathies (TSE), their rarity revealed by the fact that the incidence of the most common human TSE, Creutzfeldt-Jakob Disease, is only 1-1.5 cases per million people in the U.S. [2]. Through many studies across decades, scientists have gathered insight into the mechanisms of how prions are able to deteriorate the brain in the context of fatal and incurable diseases.
What is a Prion?
The first step to understanding prion diseases is understanding the prion. Simply put, prions are misfolded transmissible proteins that cause diseases. Proteins are not just some mysterious food you eat to grow muscles; they are complex molecules present in every part of your body, including the brain, and coordinate and control our biological processes. Composed of a chain of smaller molecules known as amino acids, proteins take cues from their environment to fold into specific shapes. There is typically a single shape, called a conformation, that a protein can take on in order to be stable and functional. Included in this shape are different structural components such as beta pleated sheets and alpha helices. While we expect one stable shape, other stable conformations can exist and cause trouble.
In the brain, there is a stable and safe form of the prion protein (PrP) called PrPC which can misfold into PrPSc, the infectious prion [3]. While both are labeled prion protein, we can think of this second shape as being the actual prion that we want to avoid. Resembling a chain reaction, one misfolded protein replicates its structure onto other prion proteins in the brain. The newly misfolded proteins do the same to other prion proteins, propagating this misfolding throughout the brain. Gradually, the misfolded protein accumulates in the brain before the first symptom appears, an incubation period that can last decades [4]. We can examine how that happens by first taking a look at the structures. While PrPC’s structure is well-documented, PrPSc’s insolubility and propensity for clustering has made studying this transformation and the prion itself difficult [3]. Nevertheless, by compiling available data and modeling different conditions, scientists have been able to estimate the structure of PrPSc. While PrPC contains more of the secondary structure known as alpha helices, PrPSc has more parallel strands in its shape. Some scientists propose that there is a sequential formation of these strands, where one newly formed strand acts as a template for the formation of the next. While a strand is not inherently worse than a helix, the change in the overall shape causes the protein to aggregate. Variations in conformation are currently thought to be responsible for different prion strains [3]. The accumulation of the protein between neurons in the brain, is one of the diagnostic criteria for prion diseases like variant Creutzfeldt-Jakob Disease [1]. Associated with these large observable changes is the conversion of the tiny protein PrPC to it’s infectious and aggregating version, PrPSc.
One way of approaching the question of how aggregation occurs is to look at the actual process of folding, rather than just the first and last step. Protein folding occurs in an instant, but it is not a leap from one state to another. A study comparing PrPC and a mutated PrP protein that is implicated in genetically acquired prion disease found a relationship between an intermediate step, a shape of the protein between the initial and final states, and the clustering of prions [5]. The same researchers designed antibodies that could suppress the structural fluctuations that lead to the formation of the intermediate, showing the significance of the discovery [5]. Studying the steps between the natural PrPC and disease-causing PrPSc is critical in understanding the mechanism of aggregation and subsequent manifestation as pathological symptoms.
How Does Prion Brain Degeneration Happen?
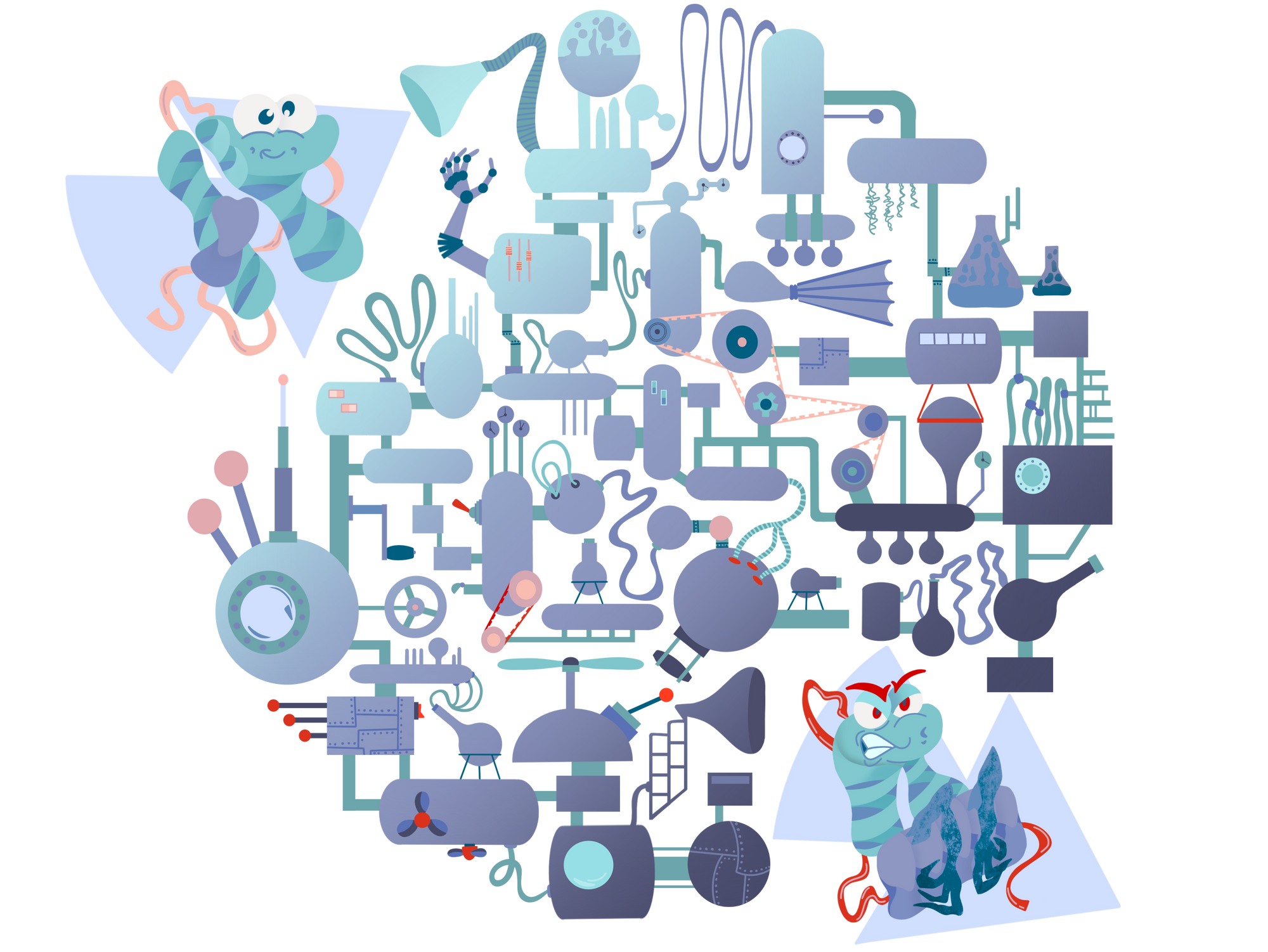
So far, we have established that good prions become harmful prions in the presence of other harmful proteins. The chain reaction will continue via peripheral nerves, such as those in the gastrointestinal tract if you ate the prion, at the site of the initial exposure all the way to your brain [4]. But why does that make us sick? Two initial ideas that come to mind are that either the decrease of the normal protein disrupts some important functions or the accumulation of the misfolded protein is toxic. For the former, a 20-year-old study in which PrP expression was halted in mice after a certain developmental stage suggested that this was not the case [6]. The mice were still alive without the presence of PrP, demonstrating that this protein might not always be necessary [6]. Therefore, although the interference of other variables cannot be ruled out, the second option becomes more likely: the aggregation of PrPSc leads to the symptoms.
While the exact mechanisms of how the increase in PrPSc results in illness are unclear, there are numerous theories. Clinically, prion diseases present themselves in different ways in the brain, but the more frequent types are characterized by spongiform degeneration, the presence of holes among neurons, reminiscent of a sponge [7]. The molecular cause of the formation of these holes, vacuolation, is largely unknown, but is being studied in relation to the disruption of specific processes. For example, the accumulation of PrPSc is assumed to cause chronic stress to the endoplasmic reticulum, where protein folding typically occurs, as it attempts to fix the misfolded proteins [8]. Through a cascade of events, this stress could cause the depletion of an enzyme that is part of the maturation control of another organelle called the lysosome, enlarging the existing organelles and giving the appearance of holes. This is the theory of one group of scientists who noted that the enzyme depletion was a universal phenomenon among the brains of prion-infected humans, mice, and cell-lines [8]. Every piece of information about specific processes and enzymes involved in the formation of spongy brains can provide hints about what to target when treating prion diseases and potentially other illnesses as well.
Potential Relations Between Prions and Alzheimer’s Disease
While prion diseases are incurable and fatal, they are fortunately very rare. In contrast, other neurodegenerative diseases are not. One such disease, Alzheimer’s Disease (AD), is one of the most frequent causes of dementia. Two proteins associated with the disease are amyloid β, which accumulates into structures called plaques, and tau, which forms tangles in brain cells [9]. Both of these structures can be thought of as harmful clusters of proteins. While AD is not infectious, AD does exhibit several qualities that resemble infectious prions.
Amyloid β specifically, like PrP, is extracellular and has been observed to have the prion-like characteristics of self-propagation and aggregation [10]. For example, Aβ, collected from an AD patient’s brain, administered into the bloodstream of mice caused the build-up of Aβ in brain blood vessels [11]. Therefore, some transmissibility of the protein is seen intravenously. Additionally, an analysis of the post-mortem brains of patients who, in life, were treated with a hormone contaminated with Creutzfeldt Jakob disease also showed Amyloid β deposition characteristic of AD [12]. This suggests that after an initial exposure, the protein propagated in the brain. While not contagious, AD spreading through certain medical practices could be possible [12]. Since then, more studies have achieved similar results [11]. These findings of shared characteristics can lend insight into both prions and Alzheimer’s Disease.
By observing how the seeding or introduction of a protein in a prion-like manner induces propagation of the protein in the brain, we can better understand methods for inhibiting this seeding and treating the disease. The protein tau, present in AD and tauopathies—diseases similar to AD, but generally not involving Aβ—spreads between neurons in this way. One study successfully designed inhibitors that could be administered into cells and block the seeding of tau and therefore stop the spread of the disease in brain tissue samples [13]. Increased understanding of how prions seed and propagate throughout the brain could be accompanied by a better grasp of the similar mechanisms in other neurodegenerative diseases. Therefore, many recent studies have explored how illnesses like Alzheimer’s and Parkinson’s exhibit prion-like characteristics [10]. The importance of this can be seen in its extension to developing therapeutics.
Where Is Research Heading?
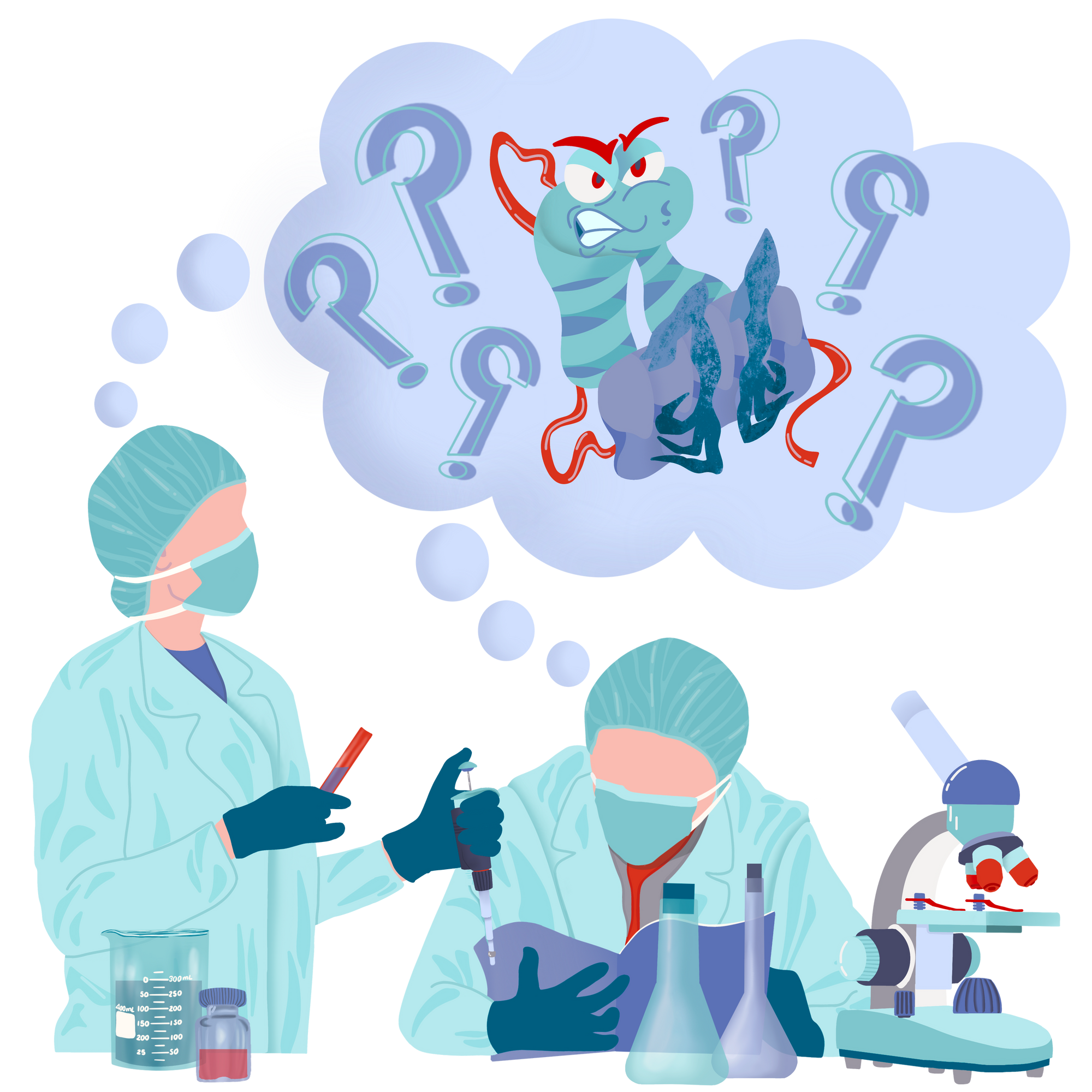
While all these studies are interesting on their own, what we often search for is how to apply this knowledge to better treat diseases. Over the past decades, many treatment possibilities have been studied, but none have shown clear results. One potential pathway for treating prion disease is to prevent the transformation of PrPC into its pathogenic form. Creating a more accurate model of the toxic PrPSc protein may bring scientists one step closer to solutions that involve designing molecules to directly target and inhibit the protein [2]. Building on previous prion studies, researchers demonstrated how a PrP suppressing treatment was able to extend the survival of prion-infected mice across a variety of models [13]. However, due to the rarity of prion disease, controlled trials are difficult to perform on humans and current treatments still center on alleviating symptoms rather than pathology itself.
There is a long way to go in the study of prions before an effective treatment is uncovered, and our knowledge of brain degeneration still has many gaps. Protein misfolding is the root cause of it, but how such tiny molecules are able to manifest themselves as macroscopic damage in the brain is difficult to explain. The better we are able to do so, the more we can potentially increase our understanding of other neurodegenerative diseases. Uncovering the secrets of prions will be a difficult mission, but the possibilities for life-saving technologies using that knowledge inspire further study into the world of transmissible proteins that make cows go mad.
References
- Diagnostic criteria. (2018, December 3). Centers for Disease Control and Prevention. https://www.cdc.gov/prions/vcjd/diagnostic-criteria.html
- Occurrence and transmission. (2019, May 8). https://www.cdc.gov/prions/cjd/occurrence-transmission.html
- Spagnolli, G., Rigoli, M., Orioli, S., Sevillano, A. M., Faccioli, P., Wille, H., Biasini, E., & Requena, J. R. (2019). Full atomistic model of prion structure and conversion. PLOS Pathogens, 15(7), e1007864. https://doi.org/10.1371/journal.ppat.1007864
- Classic CJD versus variant CJD. (2021, September 7). Centers for Disease Control and Prevention. https://www.cdc.gov/prions/cjd/classic-variant.html
- Sanz-Hernández, M., Barritt, J. D., Sobek, J., Hornemann, S., Aguzzi, A., & De Simone, A. (2021). Mechanism of misfolding of the human prion protein revealed by a pathological mutation. Proceedings of the National Academy of Sciences, 118(12), e2019631118. https://doi.org/10.1073/pnas.2019631118
- Mallucci, G. R., Ratté, S., Asante, E. A., Linehan, J., Gowland, I., Jefferys, J. G., & Collinge, J. (2002). Post-natal knockout of prion protein alters hippocampal CA1 properties, but does not result in neurodegeneration. The EMBO journal, 21(3), 202–210. https://doi.org/10.1093/emboj/21.3.202
- Letourneau-Guillon, L., Wada, R., & Kucharczyk, W. (2012). Imaging of prion diseases. Journal of Magnetic Resonance Imaging, 35(5), 998-1012. https://doi.org/10.1002/jmri.23504
- Lakkaraju, A., Frontzek, K., Lemes, E., Herrmann, U., Losa, M., Marpakwar, R., & Aguzzi, A. (2021). Loss of PIKfyve drives the spongiform degeneration in prion diseases. EMBO molecular medicine, 13(9), e14714. https://doi.org/10.15252/emmm.202114714
- Tiwari S, Atluri V, Kaushik A, Yndart A, Nair M. Alzheimer's disease: pathogenesis, diagnostics, and therapeutics. Int J Nanomedicine. 2019 Jul 19;14:5541-5554. doi: 10.2147/IJN.S200490. PMID: 31410002; PMCID: PMC6650620.
- Jaunmuktane, Z., & Brandner, S. (2020). Invited Review: The role of prion-like mechanisms in neurodegenerative diseases. Neuropathology and applied neurobiology, 46(6), 522–545. https://doi.org/10.1111/nan.12592
- Burwinkel, M., Lutzenberger, M., Heppner, F. L., Schulz-Schaeffer, W., & Baier, M. (2018). Intravenous injection of beta-amyloid seeds promotes cerebral amyloid angiopathy (CAA). Acta neuropathologica communications, 6(1), 23. https://doi.org/10.1186/s40478-018-0511-7
- Jaunmuktane, Z., Mead, S., Ellis, M., Wadsworth, J. D., Nicoll, A. J., Kenny, J., Launchbury, F., Linehan, J., Richard-Loendt, A., Walker, A. S., Rudge, P., Collinge, J., & Brandner, S. (2015). Evidence for human transmission of amyloid-β pathology and cerebral amyloid angiopathy. Nature, 525(7568), 247-250. https://doi.org/10.1038/nature15369
- Seidler, P. M., Boyer, D. R., Murray, K. A., Yang, T. P., Bentzel, M., Sawaya, M. R., Rosenberg, G., Cascio, D., Williams, C. K., Newell, K. L., Ghetti, B., DeTure, M. A., Dickson, D. W., Vinters, H. V., & Eisenberg, D. S. (2019). Structure-based inhibitors halt prion-like seeding by Alzheimer’s disease–and tauopathy–derived brain tissue samples. Journal of Biological Chemistry, 294(44), 16451-16464. https://doi.org/10.1074/jbc.ra119.009688