Imagine the brain as an epic house party with various cell types acting as partygoers, each contributing to the chaotic buzz. Amid the lively crowd, the neurons are the center of attention, setting the mood and sparking conversations with their electrical and chemical signals. Astrocytes revitalize the atmosphere with nourishing snacks and refreshments, ensuring that houseguests are hydrated and stomachs are satisfied. Meanwhile, oligodendrocytes, the DJs, keep things connected and fast by insulating everyone with music.
Then there are microglia, the unassuming, multitasking friends who play a crucial role in keeping the party going. They refill the snack bowls, clean up the spills, and keep an eye on the guests who've had too much to drink. As important as their role is, the microglia have been chronically understudied since their discovery in 1919, with the majority of research focusing on their neuronal neighbors. Over the past decade, however, these versatile cells have gained increasing prominence in neuroscience research, a result of several key papers that have illuminated the mechanisms of microglia. Recent publications have built on these findings and revealed how microglia maintain the brain's health, manage its neural connections, and fight injuries and infections.
In the real world, microglia are the immune cells of the central nervous system (CNS). Just as macrophages and lymphocytes fight off infection throughout the rest of the body, microglia are the brain and spinal cord’s immune responders. However, the function of microglia extends beyond fighting infection. Recent research has revealed several roles that include shaping neural circuits and synaptic pruning, both of which have potential therapeutic applications in treating neurodevelopmental and neurodegenerative diseases. For example, microglia play an important role in the development of Alzheimer’s disease, with genetic mutations in these cells significantly increasing the risk of disease onset [1]. This finding has opened new doors for therapeutics that target microglia to slow down Alzheimer’s progression.
Another role of microglia is synaptic pruning, an essential process in which the brain eliminates excess neuronal connections in order to refine and optimize the efficiency of neural circuits. Abnormalities in this pruning result in the development of autism and schizophrenia. Because of this, the study of microglia could result in therapies that improve synaptic connectivity and improve patient outcomes.
The study of microglia has unearthed a myriad of possibilities for understanding and treating neurological disorders. This article examines recent research breakthroughs that have advanced the neuroscientific community’s understanding of these cells and their therapeutic potential. Specifically, these studies focused on how microglial involvement in synaptic pruning and neural immune surveillance have kicked off new research and therapeutic ideas. With research accelerating at a rapid pace, tangible therapies will likely emerge sooner rather than later.
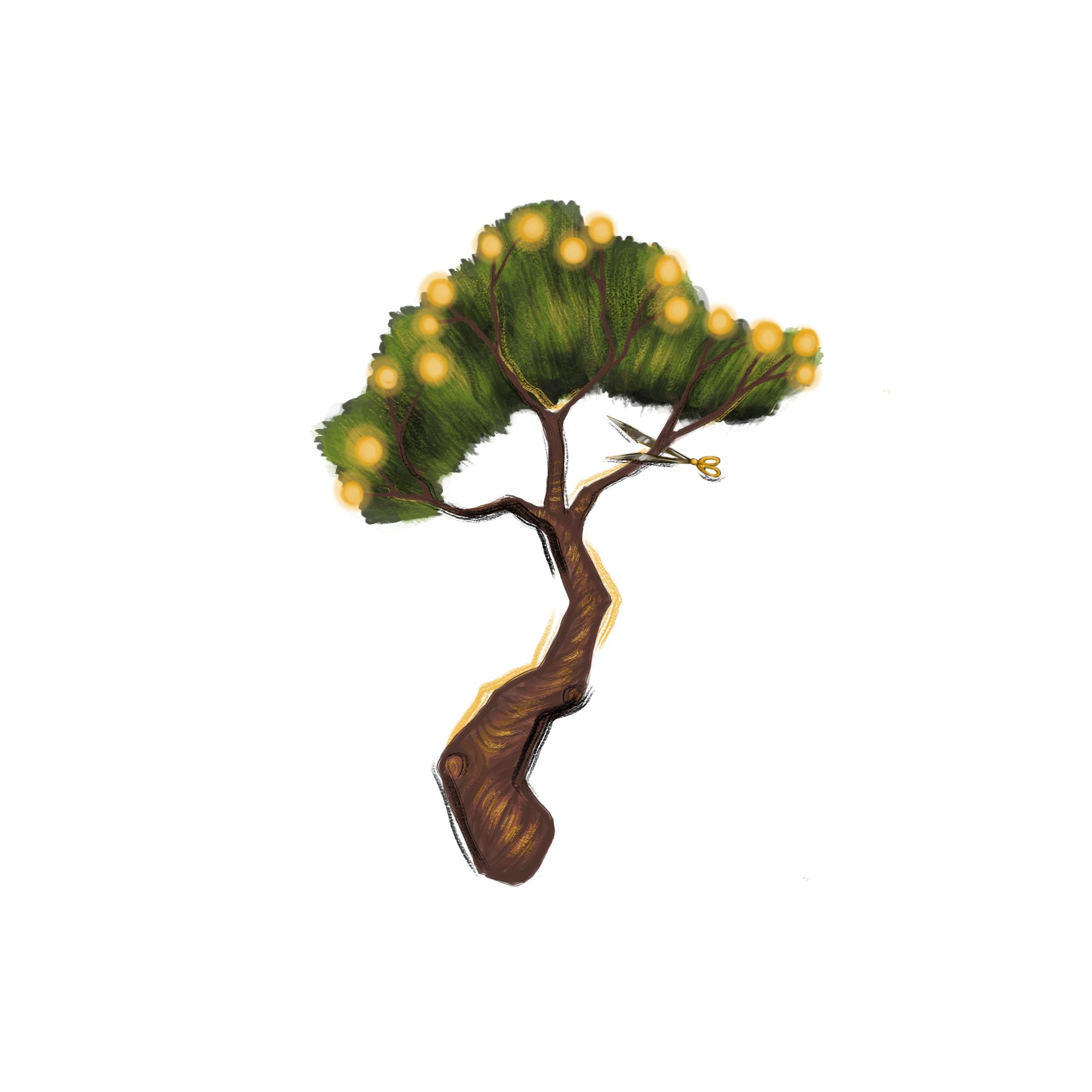
Synaptic Pruning
The developing nervous system undergoes remarkable remodeling to achieve the highly precise wiring characteristic of mature neural circuits. This precision is achieved through pruning – an extensive process in which a large subset of axons, dendrites, and synapses that initially form in abundance are eliminated over the course of development throughout the nervous system [2]. When these unnecessary synapses are identified, pruning occurs through phagocytosis by microglia. Microglia engulf the synaptic components, then digest and recycle them. The current research suggests that pruning continues into adulthood, making it an essential part of normal brain function [3].
To appreciate the significance of microglia in synaptic pruning, it is important to understand the mechanisms that drive synaptic pruning itself. A key breakthrough in understanding synaptic pruning occurred in 2007, when scientists at Stanford University discovered that the classical complement pathway has a critical role in eliminating synapses during human development [4]. The complement pathway is a pathway of proteins that initiate the activation of the complement system. The complement system, in turn, forms a highly intricate network of proteins that enhances the innate immune system's ability to target pathogens and generate inflammation. In this study, the authors revealed that C1q and C3, protein messengers of the complement pathway, aggregate near synapses and are necessary for synaptic pruning in the developing mouse retina [4].
European scientists expanded on this groundbreaking work in 2011 by offering evidence that microglia are the agents that carry out complement-mediated synaptic pruning [5]. In the developing mouse brain, they observed that microglia proliferated by dividing after activation of the Cx3cr1 protein receptor located on their cell surface. While Cx3cr1 is responsible for the abundance of microglia, these researchers hypothesized that the targeting of microglia to synapses occurs through a process involving the interaction between other complement proteins and their corresponding receptors on microglial cells. Namely, the C1q and C3 components of the complement system bind to receptors on microglia and activate pathways that result in engulfing and eliminating synapses [5]. The following year, in a continuation of their previous work, the researchers at Stanford University further illuminated the role of microglia in synaptic pruning by showing that the microglial engulfment of synapses was activity-dependent [6]. In other words, microglia can preferentially eliminate synapses with low activity because higher levels of complement proteins surround them. This finding suggests that microglial-mediated synaptic pruning is a highly regulated and selective process that refines neural circuits based on their functional activity.
While the elimination of excess synapses is essential for the proper development and function of neural circuits, abnormal synaptic pruning by microglia can lead to several neurological disorders. Overpruning, or the excessive elimination of synapses, has been implicated in the development of diseases such as Alzheimer's and schizophrenia. For example, in an Alzheimer’s mouse model, researchers demonstrated that increased microglia-mediated synaptic pruning occurred in the early stages of the disease [1]. They found that microglia, along with the complement component C1q, were excessively recruited to synapses within mouse brains, leading to increased synaptic loss and cognitive decline. This study highlighted a previously unrecognized role for microglia in the progression of Alzheimer’s, suggesting that targeting microglial activation or the complement pathway might be a promising therapeutic strategy for the disease [1].
Similarly, excessive synaptic pruning by microglia has been implicated in the development of schizophrenia. A landmark study by Harvard University researchers in 2016 identified a genetic link between the complement component C4 and the risk of developing schizophrenia [7]. They showed that increased expression of C4, which marks synapses for elimination, led to enhanced synaptic pruning in the brains of mice during postnatal development. This finding provides a mechanistic explanation for the reduced synaptic density observed in the brains of individuals with schizophrenia and further emphasizes the importance of precise regulation of synaptic pruning in maintaining proper brain function [7].
On the other hand, insufficient synaptic pruning by microglia, or underpruning, could also contribute to the development of neurological disorders. Both reduced and excessive pruning can impair the brain's excitatory versus inhibitory (E/I) balance. The brain's E/I balance refers to the balance between excitatory signals which stimulate the brain and inhibitory signals which calm the brain. Instabilities in this network of communication are a possible mechanism for the progression of neurodevelopmental disorders such as autism spectrum disorder (ASD) [8]. In 2014, Columbia University researchers provided evidence linking reduced synaptic pruning during development to ASD [9]. They observed increased dendritic spine density in ASD specimens. Dendritic spines are tiny protrusions from a neuron's dendrite where synapses occur. An increase in dendritic spine density could suggest an abundance of synaptic connections, which could be indicative of underpruning. Compared to a control group, spine density in the adolescent ASD group was significantly higher, suggesting that developmental pruning of excitatory synapses is reduced in ASD patients. Further research is needed to determine the extent to which impairments in synaptic pruning and microglia-mediated synapse engulfment are involved in ASD. Mouse models of obsessive-compulsive disorder (OCD) and Rett syndrome, which exhibit behavioral phenotypes similar to ASD, have reported similar deficits in synaptic pruning, suggesting that these findings may also be applicable to these conditions [10][11].
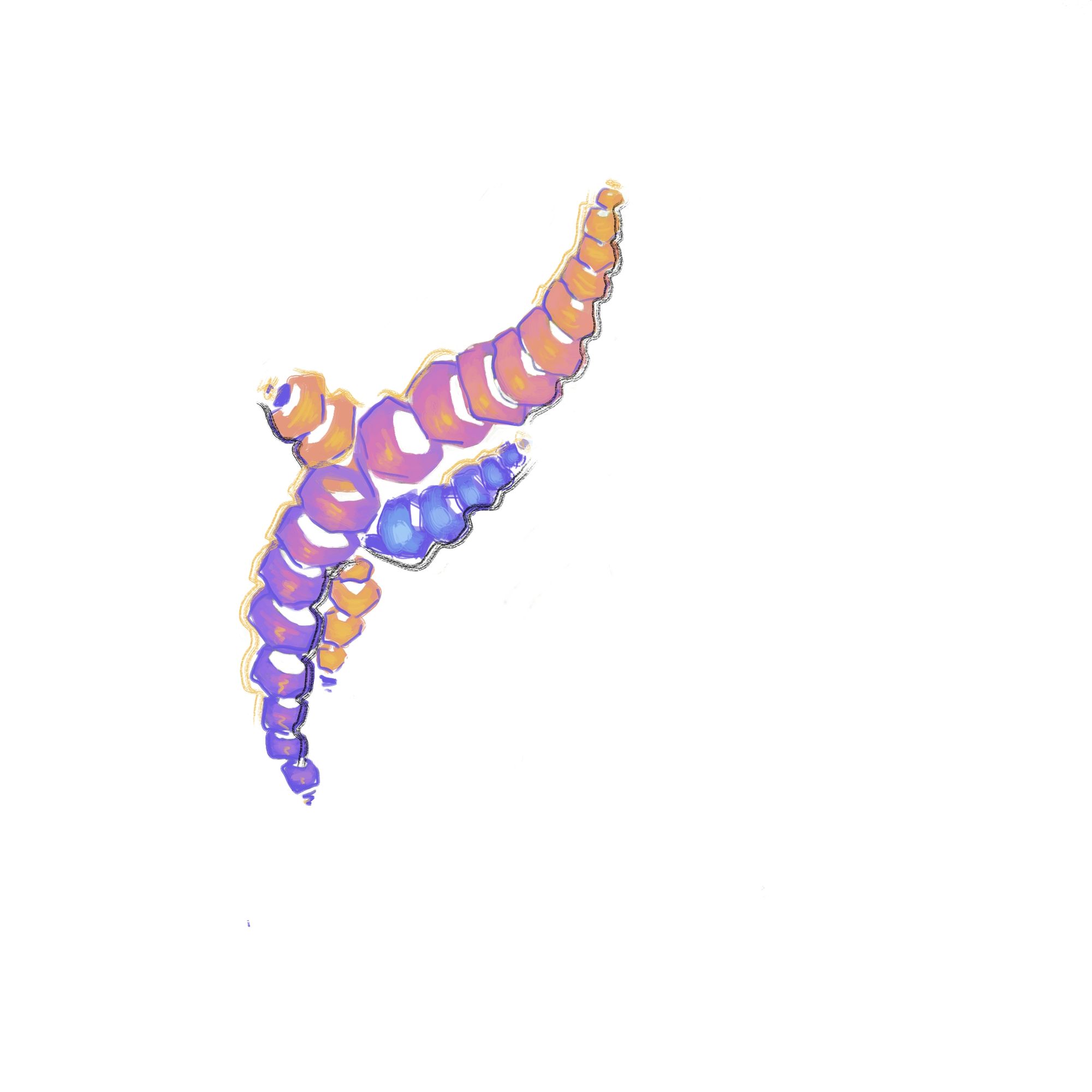
Immune Function
The immune system of the central nervous system is distinct from that of the peripheral immune system, primarily due to the unique nature of the blood-brain barrier (BBB) and the specialized immune cells that reside within the brain [12]. The BBB is a selective barrier composed of tightly connected endothelial cells and astrocytes [13]. This barrier protects the CNS from harmful pathogens, but also prevents immune cells, including T-cells, from entering the brain. Because some pathogens have evolved strategies to enter the CNS through the BBB, the brain requires its own dedicated immune system. A small number of T cells can be found in the CNS under normal conditions, but they are not ideal for fighting pathogens because they can set off inflammatory cascades that result in unintentional collateral damage to neural tissue [14]. Instead, the immune system relies on microglia, which remain within the CNS throughout an individual’s entire life. Microglia are best suited for neural immune surveillance due to their ability to digest pathogens while maintaining minimal disruption to neural function [15].
Microglia possess a range of mechanisms to detect, engage, and eliminate bacteria that have managed to infiltrate the CNS. These specialized immune cells express various pattern recognition receptors (PRRs) that allow them to recognize proteins present on the surface of bacteria [16]. Upon detection of these patterns, microglia become activated, undergoing morphological and functional changes that enable them to combat the invading pathogens [16]. One key mechanism employed by microglia to remove bacteria is phagocytosis. Through phagocytosis, microglia engulf and internalize bacteria within specialized compartments called phagosomes, which then fuse with lysosomes containing enzymes and antimicrobial substances. This creates phagolysosomes, in which bacteria are degraded and eliminated of the bacteria [17]. Microglia also can secrete cytokines and chemokines to recruit and lead other immune cells, such as neutrophils, across the BBB to aid in the clearance of bacteria [17].
However, microglial defenses can occasionally be insufficient due to the evasion strategies employed by certain pathogens. These insufficiencies are responsible for the development of bacterial meningitis, among other diseases. For example, pathogens like Streptococcus pneumoniae and Neisseria meningitidis have developed mechanisms to circumvent microglial detection and clearance [18]. Streptococcus pneumoniae can produce a capsule composed of polysaccharides, which masks the bacterial surface and prevents recognition by microglial PRRs. Neisseria meningitidis can evade the immune response by mimicking host cells, expressing proteins on its surface that are similar to those found on human cells, thereby avoiding detection by microglia [18]. Additionally, some pathogens can exploit the host's immune response, leading to a strong inflammatory response that can cause damage to brain tissue and the BBB itself. For instance, Streptococcus pneumoniae is capable of inducing the production of pro-inflammatory cytokines which can lead to increased BBB permeability and facilitate the invasion of additional pathogens and immune cells into the CNS [19]. This inflammatory response further exacerbates damage to brain tissue.
These new understandings about the role of microglia in the brain’s response to bacteria have led to therapeutic applications that are already being tested. Pneumococcal meningitis is typically treated with ceftriaxone, a “bacteriolytic” antibiotic that destroys the bacteria and leads to the release of bacterial components like the cell wall. This process can also trigger further activation of microglia and promote neuroinflammation [20]. As a result, researchers have begun to explore the use of potent but non-bacteriolytic antibiotics such as daptomycin. This agent has shown potential in experimental models of pneumococcal meningitis, effectively killing bacteria and mitigating neuroinflammation. However, daptomycin's utility is limited as it cannot penetrate the outer membrane of Gram-negative bacteria, restricting its bactericidal activity to Gram-positive bacteria only [21]. Consequently, two recent studies have explored the combination of non-bacteriolytic antibiotics with ceftriaxone treatment, with both reporting improved neurofunctional outcomes in experimental models of pneumococcal meningitis [22]. This suggests that tailored combinations of therapies may offer improved outcomes in treating this debilitating disease.
The development of Cruetzfeldt-Jakob disease, a disease caused by the accumulation of misfolded proteins called prions, also highlights microglial shortcomings. In healthy people, bacteria and viruses display cell surface receptors that can be identified by microglia, leading to an effective response. In contrast, prions are composed of misfolded host proteins called PrPSc, derived from the normal cellular prion protein (PrPC) [23]. The structural similarity between PrPSc and PrPC makes it difficult for microglia to differentiate between the two. As a result, PrPSc accumulates and forms plaques that cause damage to the brain. Not only do microglia fail to remove PrPSc proteins, but chronic microglial activation results in excess inflammation in the brain [24]. When encountering prions, microglia produce pro-inflammatory cytokines and reactive oxygen species that cause damage to neurons. The balance between protective and detrimental microglial functions is disrupted, leading to chronic neuroinflammation and exacerbation of the disease [24].
Researchers have already begun investigating therapies for prion diseases through the lens of microglial function. Activated microglia adopt different phenotypes depending on the environment they are in. In the context of prion diseases, microglia switch between one form with enhanced phagocytosis and anti-inflammatory factors and another pro-inflammatory and neurotoxic form [25]. Thus, a potential therapeutic avenue includes guiding microglia towards a phenotype that efficiently phagocytoses prions while secreting anti-inflammatory factors. This would avoid the neurotoxicity that typically occurs when chronically active microglia secrete inflammatory agents. In animal models, modulation of microglial receptors, such as Toll-like receptors (TLRs) and CD14, which are involved in the innate immune response, has already shown potential [25].
Another potential approach focuses on targeting CSF1R signaling, a player in microglial activation and proliferation. A key inhibitor in the CSF1R signaling pathway is GW2580. In prion-infected mice, the addition of GW2580 reduced microglial proliferation, adjusted cytokine expression (proteins that mediate immune and inflammatory responses), and improved the outcomes of prion-infected mice [26]. This outcome highlights CSF1R signaling as a possible therapeutic target in prion diseases. Additionally, in one 2017 experiment, researchers found that activation of microglia with a retrovirus treatment resulted in temporary prion clearance in diseased mice [27]. This suggests that carefully controlled microglial stimulation could improve the ability of microglia to phagocytose prions. As a whole, these experiments indicate that microglial therapy holds the key to the future treatment of prion diseases.
Conclusion
The evolution of neuroscience research reveals the significant role microglia play in maintaining brain health. From synaptic pruning to neural immune surveillance, the intricacies of these cells' functions are continually being uncovered. Their pivotal role in synaptic pruning points to therapeutic potential for conditions like Alzheimer's, schizophrenia, and ASD, while their contribution to immune defense opens doors for innovative treatments for meningitis and prion diseases. The burgeoning exploration into microglial biology offers promising prospects, propelling us toward tangible therapies for various neurological disorders. As research accelerates, the once-understudied microglia are poised to take center stage in neuroscience.
References
- Hong, S., Beja-Glasser, V. F., Nfonoyim, B. M., Frouin, A., Li, S., Ramakrishnan, S., Merry, K. M., Shi, Q., Rosenthal, A., Barres, B. A., Lemere, C. A., Selkoe, D. J., & Stevens, B. (2016). Complement and microglia mediate early synapse loss in Alzheimer mouse models. Science (New York, N.Y.), 352(6286), 712–716. doi.org/10.1126/science.aad8373
- Faust, T. E., Gunner, G., & Schafer, D. P. (2021). Mechanisms governing activity-dependent synaptic pruning in the developing mammalian CNS. Nature reviews. Neuroscience, 22(11), 657–673. doi.org/10.1038/s41583-021-00507-y
- Gonçalves, J. T., Bloyd, C. W., Shtrahman, M., Johnston, S. T., Schafer, S. T., Parylak, S. L., Tran, T., Chang, T., & Gage, F. H. (2016). In vivo imaging of dendritic pruning in dentate granule cells. Nature neuroscience, 19(6), 788–791. doi.org/10.1038/nn.4301
- Stevens, B., Allen, N. J., Vazquez, L. E., Howell, G. R., Christopherson, K. S., Nouri, N., Micheva, K. D., Mehalow, A. K., Huberman, A. D., Stafford, B., Sher, A., Litke, A. M., Lambris, J. D., Smith, S. J., John, S. W., & Barres, B. A. (2007). The classical complement cascade mediates CNS synapse elimination. Cell, 131(6), 1164–1178. doi.org/10.1016/j.cell.2007.10.036
- Paolicelli, R. C., Bolasco, G., Pagani, F., Maggi, L., Scianni, M., Panzanelli, P., Giustetto, M., Ferreira, T. A., Guiducci, E., Dumas, L., Ragozzino, D., & Gross, C. T. (2011). Synaptic pruning by microglia is necessary for normal brain development. Science (New York, N.Y.), 333(6048), 1456–1458. doi.org/10.1126/science.1202529
- Schafer, D. P., Lehrman, E. K., Kautzman, A. G., Koyama, R., Mardinly, A. R., Yamasaki, R., Ransohoff, R. M., Greenberg, M. E., Barres, B. A., & Stevens, B. (2012). Microglia sculpt postnatal neural circuits in an activity and complement-dependent manner. Neuron, 74(4), 691–705. doi.org/10.1016/j.neuron.2012.03.026
- Sekar, A., Bialas, A. R., de Rivera, H., Davis, A., Hammond, T. R., Kamitaki, N., Tooley, K., Presumey, J., Baum, M., Van Doren, V., Genovese, G., Rose, S. A., Handsaker, R. E., Schizophrenia Working Group of the Psychiatric Genomics Consortium, Daly, M. J., Carroll, M. C., Stevens, B., & McCarroll, S. A. (2016). Schizophrenia risk from complex variation of complement component 4. Nature, 530(7589), 177–183. doi.org/10.1038/nature16549
- Gatto, C. L., & Broadie, K. (2010). Genetic controls balancing excitatory and inhibitory synaptogenesis in neurodevelopmental disorder models. Frontiers in synaptic neuroscience, 2, 4. doi.org/10.3389/fnsyn.2010.00004
- Tang, G., Gudsnuk, K., Kuo, S. H., Cotrina, M. L., Rosoklija, G., Sosunov, A., Sonders, M. S., Kanter, E., Castagna, C., Yamamoto, A., Yue, Z., Arancio, O., Peterson, B. S., Champagne, F., Dwork, A. J., Goldman, J., & Sulzer, D. (2014). Loss of mTOR-dependent macroautophagy causes autistic-like synaptic pruning deficits. Neuron, 83(5), 1131–1143. doi.org/10.1016/j.neuron.2014.07.040
- Chen, S. K., Tvrdik, P., Peden, E., Cho, S., Wu, S., Spangrude, G., & Capecchi, M. R. (2010). Hematopoietic origin of pathological grooming in Hoxb8 mutant mice. Cell, 141(5), 775–785. doi.org/10.1016/j.cell.2010.03.055
- Derecki, N. C., Cronk, J. C., Lu, Z., Xu, E., Abbott, S. B., Guyenet, P. G., & Kipnis, J. (2012). Wild-type microglia arrest pathology in a mouse model of Rett syndrome. Nature, 484(7392), 105–109. doi.org/10.1038/nature10907
- Dantzer R. (2018). Neuroimmune Interactions: From the Brain to the Immune System and Vice Versa. Physiological reviews, 98(1), 477–504. doi.org/10.1152/physrev.00039.2016
- Mapunda, J. A., Tibar, H., Regragui, W., & Engelhardt, B. (2022). How Does the Immune System Enter the Brain? Frontiers in immunology, 13, 805657. doi.org/10.3389/fimmu.2022.805657
- Schetters, S. T. T., Gomez-Nicola, D., Garcia-Vallejo, J. J., & Van Kooyk, Y. (2018). Neuroinflammation: Microglia and T Cells Get Ready to Tango. Frontiers in immunology, 8, 1905. doi.org/10.3389/fimmu.2017.01905
- Yang, I., Han, S. J., Kaur, G., Crane, C., & Parsa, A. T. (2010). The role of microglia in central nervous system immunity and glioma immunology. Journal of clinical neuroscience: official journal of the Neurosurgical Society of Australasia, 17(1), 6–10. doi.org/10.1016/j.jocn.2009.05.006
- Saijo, K., Crotti, A., & Glass, C. K. (2013). Regulation of microglia activation and deactivation by nuclear receptors. Glia, 61(1), 104–111. doi.org/10.1002/glia.22423
- Janda, E., Boi, L., & Carta, A. R. (2018). Microglial Phagocytosis and Its Regulation: A Therapeutic Target in Parkinson's Disease? Frontiers in molecular neuroscience, 11, 144. doi.org/10.3389/fnmol.2018.00144
- Thorsdottir, S., Henriques-Normark, B., & Iovino, F. (2019). The Role of Microglia in Bacterial Meningitis: Inflammatory Response, Experimental Models and New Neuroprotective Therapeutic Strategies. Frontiers in microbiology, 10, 576. doi.org/10.3389/fmicb.2019.00576
- Yau, B., Hunt, N. H., Mitchell, A. J., & Too, L. K. (2018). Blood‒Brain Barrier Pathology and CNS Outcomes in Streptococcus pneumoniae Meningitis. International journal of molecular sciences, 19(11), 3555. doi.org/10.3390/ijms19113555
- Liechti, F. D., Grandgirard, D., & Leib, S. L. (2015). Bacterial meningitis: insights into pathogenesis and evaluation of new treatment options: a perspective from experimental studies. Future microbiology, 10(7), 1195–1213. doi.org/10.2217/fmb.15.43
- Randall, C. P., Mariner, K. R., Chopra, I., & O'Neill, A. J. (2013). The target of daptomycin is absent from Escherichia coli and other gram-negative pathogens. Antimicrobial agents and chemotherapy, 57(1), 637–639. doi.org/10.1128/AAC.02005-12
- Klein, M., Höhne, C., Angele, B., Högen, T., Pfister, H. W., Tüfekci, H., & Koedel, U. (2019). Adjuvant non-bacteriolytic and anti-inflammatory combination therapy in pneumococcal meningitis: an investigation in a mouse model. Clinical microbiology and infection: the official publication of the European Society of Clinical Microbiology and Infectious Diseases, 25(1), 108.e9–108.e15. doi.org/10.1016/j.cmi.2018.03.039
- Aguzzi, A., & Zhu, C. (2017). Microglia in prion diseases. The Journal of clinical investigation, 127(9), 3230–3239. doi.org/10.1172/JCI90605
- Srivastava, S., Katorcha, E., Makarava, N., Barrett, J. P., Loane, D. J., & Baskakov, I. V. (2018). Inflammatory response of microglia to prions is controlled by sialylation of PrPSc. Scientific reports, 8(1), 11326. doi.org/10.1038/s41598-018-29720-z
- Ertürk, A., Mentz, S., Stout, E. E., Hedehus, M., Dominguez, S. L., Neumaier, L., Krammer, F., Llovera, G., Srinivasan, K., Hansen, D. V., Liesz, A., Scearce-Levie, K. A., & Sheng, M. (2016). Interfering with the Chronic Immune Response Rescues Chronic Degeneration After Traumatic Brain Injury. The Journal of neuroscience: the official journal of the Society for Neuroscience, 36(38), 9962–9975. doi.org/10.1523/JNEUROSCI.1898-15.2016
- Olmos-Alonso, A., Schetters, S. T., Sri, S., Askew, K., Mancuso, R., Vargas-Caballero, M., Holscher, C., Perry, V. H., & Gomez-Nicola, D. (2016). Pharmacological targeting of CSF1R inhibits microglial proliferation and prevents the progression of Alzheimer's-like pathology. Brain: a journal of neurology, 139(Pt 3), 891–907. doi.org/10.1093/brain/awv379
- Muth, C., Schröck, K., Madore, C., Hartmann, K., Fanek, Z., Butovsky, O., Glatzel, M., & Krasemann, S. (2017). Activation of microglia by retroviral infection correlates with transient clearance of prions from the brain but does not change incubation time. Brain pathology (Zurich, Switzerland), 27(5), 590–602. doi.org/10.1111/bpa.12441