Awareness During Anesthetized Surgery
For many, the thought of undergoing surgery can be a terrifying fear. Operations in the United States often come with an exorbitant price tag and without any guarantee of success. At the extreme end, there is even a risk of death. This last fear is perhaps amplified by the use of general anesthesia, a depressant commonly used in prolonged surgeries. General anesthesia subjects patients to a sleep-like state, causes a complete loss of sensation, and induces amnesia. The possibility of never waking up from surgery is terrifying by itself. But what can be even more frightening is experiencing intraoperative awareness, which manifests either as accidental consciousness during surgery, or explicit episodic recall afterwards. During the deepest level of sedation, patients with awareness are essentially awake but paralyzed. Immobilized individuals have no choice but to hear the unnerving clanging of surgical instruments and the discussion of doctors as the scalpel meets skin. Despite it all, the patient appears peacefully asleep to the outsider’s eye, and thus the operators continue with the procedure. Although intraoperative awareness occurs at only a 0.13% incidence rate [1], the possibility remains a point of concern among patients.
Unsurprisingly, a significant percentage of patients who experience intraoperative awareness report having depression, anxiety, and PTSD [2]. While some consciously feel the pain of surgical stimulation during the operation, others are tormented by isolated auditory recall and episodic feelings of suffocation afterwards [3]. More severe experiences of intraoperative awareness can lead to a fear of lying flat, an avoidance of future medical care, and long-term psychological distress [4]. These fears are especially understandable considering that the explicit scientific mechanisms of both general anesthesia and intraoperative awareness remain unknown. Currently, experts suggest that anesthetic awareness is caused by low anesthetic dosage, difficulties in initially administering the anesthesia, and resistance to anesthetics due to chronic use [5]. Cutting-edge research further examines the link between general anesthesia and its physiological effects by exploring the exact mechanics behind general anesthesia and contextualizing them in the well-researched topic of natural sleep.
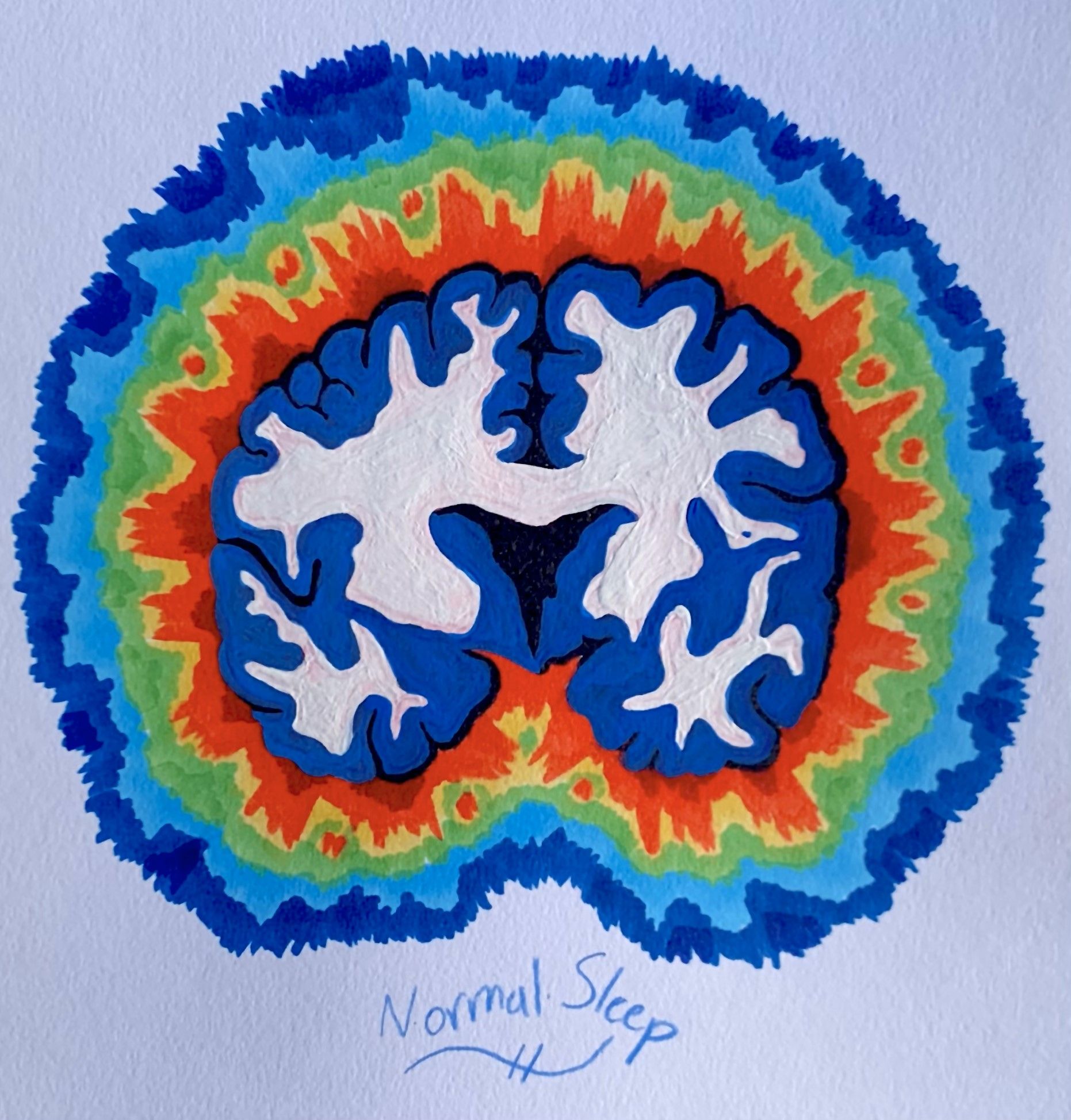
Electrical Signaling in General Anesthesia
When the injection of general anesthesia starts to take its effect, patients can be observed to succumb into a slumbering state. Signs of anxiety drift away through the IV tube, muscles relax into a limp stillness, and pain ebbs away into nothingness. Because both natural sleep and anesthesia induce a reversible state of consciousness, it is logical to compare the two. However, the two states of unconsciousness have important differences. For one, the depth of unconsciousness between sleep and anesthesia differs significantly. While individuals can easily wake up from sleep to a loud noise or a tactile stimulus, general anesthesia triggers a sustained state of behavioral unresponsiveness which cannot be disrupted even by painful stimuli. General anesthesia also keeps the body entirely immobile throughout its application, whereas natural sleep may demonstrate occasional kicking or rolling about [6]. These anesthetic inhibitions on motor activity and sensory perception are vital for the safe and effective execution of surgical operations.
The differences between sleep and anesthesia extend beyond what can be observed by the naked eye. Like many other physiological processes that repeat throughout the day, such as human heartbeat or digestion, natural sleep operates on cycles. These “Ultradian” rhythms divide sleep into two overarching categories: non-rapid eye movement (NREM) and rapid eye movement (REM) sleep. A glimpse of Ultradian patterns can be caught in the screen of an EEG, an electronic medical device which can allow doctors to study brain activity as the stages of sleep change over time. While the phases within NREM sleep typically display slow-wave electrical patterns on the EEG, REM sleep demonstrates high electrical activity. During REM sleep, a structure in the brain called the cerebral cortex stays active, allowing for dreaming and memory consolidation [7].
Comparatively, the anesthetized brain does not follow the same cycle as natural sleep. In fact, electrical recordings of a brain under general anesthesia frequently display severely reduced electrical activity, similar to that of a comatose brain. During the “maintenance period,” a stage of the operation when general anesthesia levels are maintained, electrical activity shifts into patterns resembling NREM sleep. If anesthetic levels are maintained for long enough, patients approach a flatlined “isoelectric” reading. At this extreme, there is a near-complete loss of electrical activity in the cortex. Devoid of the high-activity nature of REM sleep or the slow-wave character of NREM sleep, the isoelectric tracing allows the brain to be protected during neurosurgery and can stop certain types of seizures from occurring [8].
The electrical activity also repeats in certain distinct patterns during the anesthesia maintenance period. Observations of the deeply anesthetized brain report alternating phases of high voltage spikes and flatlining phases of no brain activity. Termed “burst suppression” by anesthesiologists, these patterns are believed to result from neuronal signaling to a highly unresponsive cortex [9]. Specifically, general anesthesia can cause the depletion of extracellular calcium levels, which interferes with the release of certain neurotransmitters that depend on calcium. Since the release of neurotransmitters propagates information from neuron to neuron, the inhibition of neurotransmitter release directly causes the “suppression” period of minimal brain activity. Correspondingly, the “burst” phase is associated with an increase in electrical activity as calcium ions are restored to normal concentrations [6,8] The absence of burst suppression patterns during sleep is a key electrophysiological difference between sleep and general anesthesia.
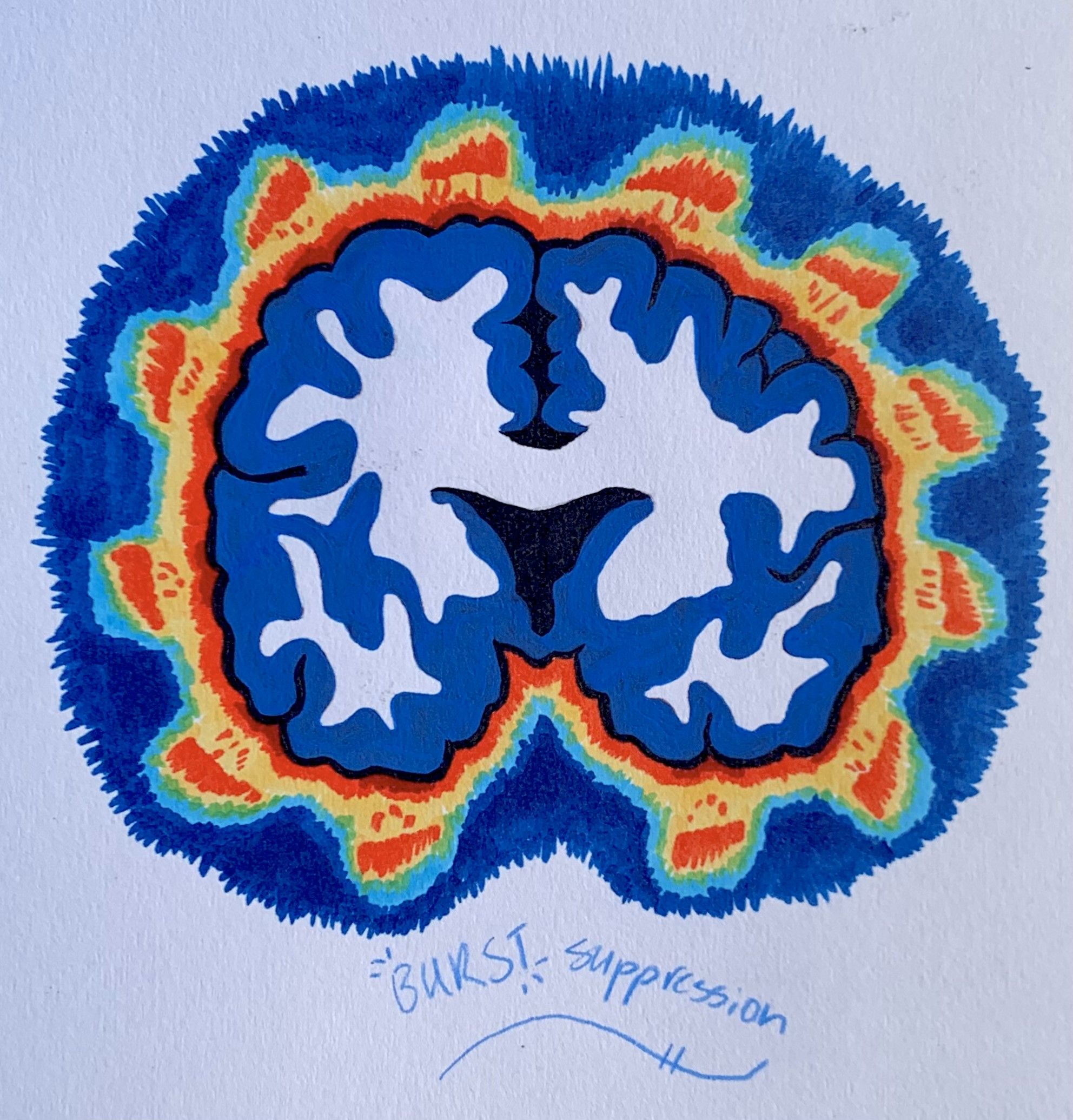
In addition to these differences, there are also anatomical differences between natural sleep and anesthesia. Sleep and anesthesia vary according to the target location of altered electrical signaling. Sleep is facilitated by a withdrawal of excitatory electrical signals in the “subcortical” area beneath the cortex. In contrast, anesthetics induce unconsciousness by attacking specific targets both in the cortex and subcortex. Different types of anesthetic drugs have different combinations of these subcortical and cortical targets. When each anesthetic acts upon its associated sites, electrical activity is generally inhibited. This inhibitory effect leads to decreased arousal when targeted at the subcortex and simultaneously degrades consciousness at the cortex [7]. Thus, an insufficient amount of electrical inhibition can lead to rare cases of intraoperative awareness. Although the exact neurobiological mechanics of intraoperative awareness still remain unclear, current research is investigating the neurophysiology of anesthetic state transitions. Researchers are accomplishing this by comparing the neuroanatomical pathways of anesthesia to observations of consciousness made during clinical practice [10].
Biochemical Agents of General Anesthesia
Despite its widespread integration into modern medicine, little is known about the true molecular mechanisms of anesthesia. Studies are particularly limited by its biophysiological complexity, which varies according to the type of anesthetic administered and the concentration injected. At low levels, anesthetics can induce overactive body responses and hypnosis. In contrast, they facilitate deep sedation and reduced motor responses to noxious stimuli at higher concentrations [11]. To further complicate things, studies on newts and mice have demonstrated reemergence from their sleeplike state when anesthesia is delivered at high pressures. These differences in the application of anesthesia and its diversity of effects increases the difficulty of researching anesthetics [12, 13]. Today, scientists struggle to pinpoint each of the affiliated chemical interactions and intertwine them to explain a coherent, larger network of physiological responses.
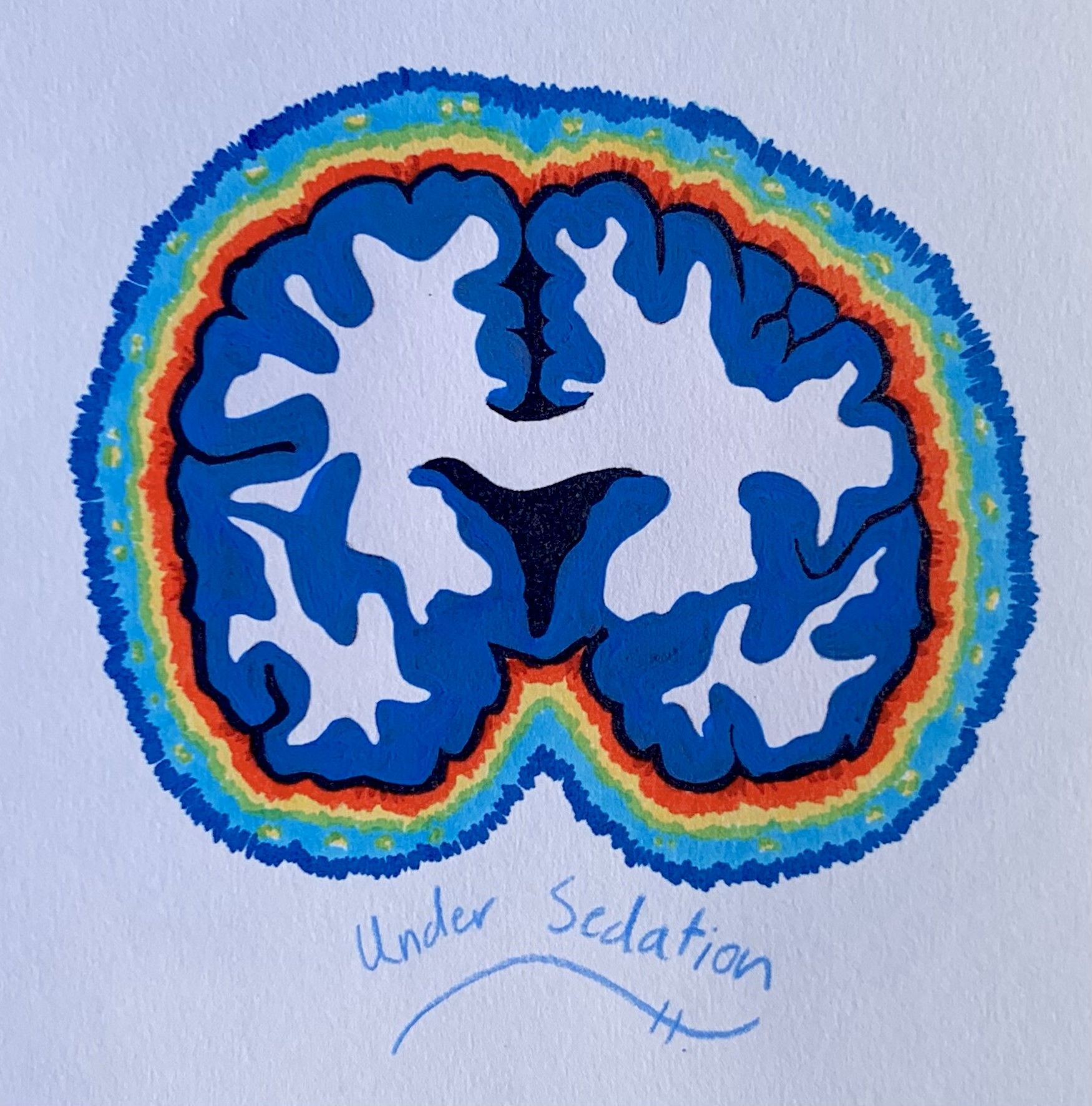
The many influential theories about the mechanisms responsible for the effects of anesthesia can be divided into two major categories: lipid theories and protein theories. Lipid theories suggest that anesthetic molecules modify the structure or activity of the cell membrane, which consequently changes the function of certain membrane-bound proteins. German pharmacologist Hans Horst Meyer and British physiologist Charles Overton independently proposed lipid theories, in which they postulated that potent anesthetics were more soluble in fats. Their lipid theory assumed that the fat-soluble properties of anesthetics in the lipid bilayer of neuronal cell membranes disrupted cellular activity, thus prompting the anesthetic effect [7]. As the twentieth century progressed, the lipid theory further evolved with the critical volume hypothesis, which proposed that anesthetics increased membrane volume and induced anesthesia by altering membrane fluidity and thickness once the anesthetic concentration passed a certain threshold value [12]. Because every neuron possesses a cell membrane that can be affected by this action, the lipid theory can be applied to the large chemical variety of general anesthetic agents. Its ideas also support the pressure reversion phenomena, which states that anesthetic molecules alter the pressure of membranes. This induces a conformational change in the membrane-bound proteins responsible for cell signaling [14]. However, experimental verification of this hypothesis is extremely difficult. As a result, research remains inconclusive in identifying the specific membrane property affected by anesthetics and the way that the associated proteins’ functions are changed.
Although the lipid theory dominated for nearly a century, a newfound observation in the 1980s revealed the interaction of general anesthetics with hydrophobic sites on proteins rather than the lipid membrane itself, leading to a paradigm shift in general anesthesia research [15]. Unlike lipid theories, many protein theories suggest that anesthetics function by interacting with the active site of certain membrane-bound proteins. However, interactions of proteins with their substrates are typically highly specific, meaning that these protein theories fail to account for the diverse chemical variety of general anesthetic substrates. To explain this issue, scientists proposed that anesthetics indirectly affected the protein structure rather than binding to the active site [12]. Today, the mechanisms of general anesthesia are widely studied by identifying the protein targets of anesthetics.
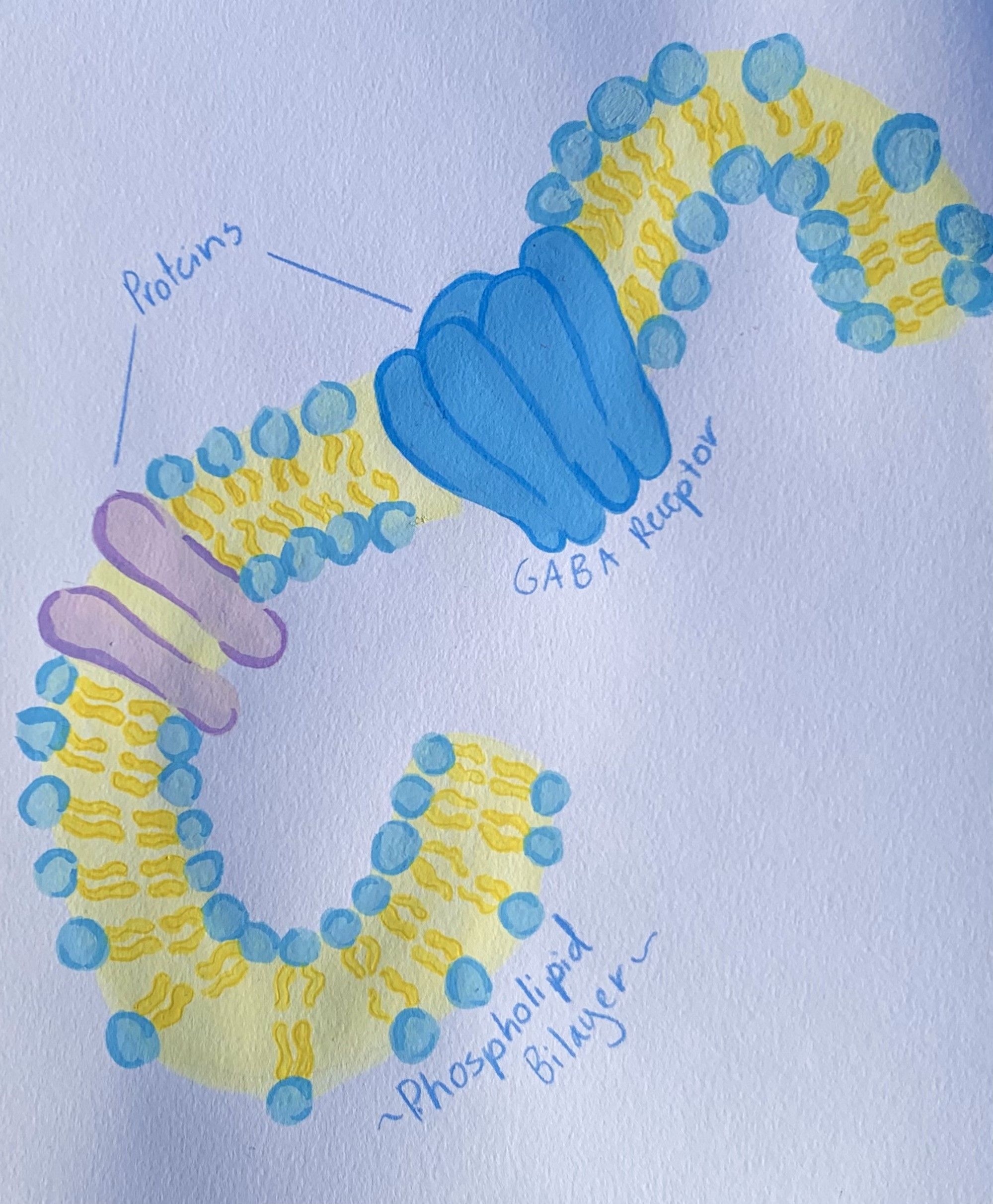
Current research largely defines ion-regulating receptors and voltage-gated ion channels as the major protein targets for general anesthetics. Considerable work has explored the interaction of anesthetics with one such target called GABAA receptors. When the GABA neurotransmitter binds to the GABAA receptor, action potentials are inhibited due to changes in the flow of ions into the cell [11]. In this way, GABAA facilitates the majority of inhibitory neurotransmission in the central nervous system. Extensive research has established GABAA as one of the well-characterized neurotransmitters responsible for natural sleep [16]. General anesthetics similarly involve the use of GABAA and induce sleep by amplifying the action of GABA neurotransmitters on GABAA receptors. At sufficiently high concentrations, the presence of anesthetics can even activate GABAA receptors without the neurotransmitter present. Like the GABA neurotransmitter, general anesthetics are able to inhibit action potentials by potentiating the ion currents responsible for inhibitory neurotransmission [17]. The inhibitory signals generated prevent the propagation of information from cell to cell, which ultimately gives rise to anesthesia [18].
Although widely studied, GABAA is not the only anesthetic target discovered. Studies reveal that many other ion channels, such as membrane-bound glycine receptors or potassium channels, also play a role in activating anesthetic effects. This is perhaps unsurprising, as the neuro depressive mechanism of sleep is also heavily regulated by the flow of ions such as potassium and calcium [19]. Like GABAA, glycine receptors affected by general anesthetics inhibit action potentials by modulating ion flow into the neuronal cell. Activation of glycine receptors is known to largely contribute to the immobile effect of anesthesia [20]. On the other hand, some types of potassium ion channels also mediate anesthesia. The flow of potassium ions through these channels generates electrical currents that determine the neuron’s resting membrane potential. When volatile anesthetics are introduced into the body, these channels are activated, causing increased potassium ion movement out of the cell. This shift in net ion movement causes a corresponding change in the cell’s resting voltage. As a result, the cell’s resting voltage is changed, and neuron activity is inhibited [21]. The accumulation of inhibitory signals leads to a widespread neurodepression across the central nervous system, ultimately producing the expected effects of general anesthesia: reduced motor activity, impaired reflexes to stimuli, and incoordination [11, 22]. Given that there are far more biochemical players involved in inducing general anesthesia, it comes as no surprise that scientists are still researching the exact mechanisms responsible.
Resurfacing: Postoperative Amnesia, Dreaming, & Sleep Debt
Upon waking up from general anesthesia, patients will find their surroundings shifted. Now inside the PACU (post-anesthesia care unit), individuals groggily wake up to the warm embrace of nurses, friends, or family. Compared to the short amount of time it takes to wake up from natural sleep, the offset of anesthesia can take hours. Many report feeling nauseous, cold, or disoriented upon reemergence, but they should have no recollection of the procedure itself. Once fully awake and pain-free, patients are discharged to return to life outside of the hospital.
Though the mechanism of how anesthesia induces amnesia is unknown, mice studies have reported that general anesthesia impairs the activity of neurons in the hippocampus, a subcortical structure responsible for learning, memory, and spatial navigation. Specifically, they inhibit long-term potentiation, the process by which the strength of signal transmission increases between neurons. The inhibition of this mechanism ultimately leads to amnesia, but the intermediate steps in between reduced hippocampal activity and amnesia have yet to be discovered [23].
An inability to recall memories of the procedure itself, however, does not imply the absence of dreaming under anesthesia. In fact, studies demonstrate that about 22% of patients report having had dreams upon reemergence from anesthesia [24]. Research shows that these dreams are mostly influenced by events that happened prior to sedation, and that dreaming typically occurs under light anesthesia. Most patients describe their dreams as short and pleasant, but a slim percentage of patients have dreams that include sensations of the procedure. This latter phenomenon is thought to occur at the edge of consciousness, near the equilibrium point between anesthesia and intraoperative awareness. The majority of dreams, though, likely occur during the recovery phase after anesthesia has been removed [25]. This common presence of dreaming may be one reason why anesthesia is often mistaken as sleep.
Given the similarities between anesthesia and sleep, one may wonder if anesthesia possesses the same ability as sleep to reverse sleep deprivation, a condition which builds up a sleep deficit called “sleep debt”. Scientists reveal that different anesthetic drugs have varying capacities for restoring sleep debt. On one hand, the injection of an anesthetic called propofol restored sleep deprivation at a rate similar to that of sleep and did not incur any additional sleep debt [26]. On the other hand, volatile anesthetics such as sevoflurane, isoflurane, and halothane all incurred a deficit in REM sleep, with halothane also accumulating NREM sleep debt. Sevoflurane in particular was also observed to reverse NREM sleep deprivation, but had no impact on restoring insufficient REM sleep [5]. The sleep debt accrued after general anesthesia strongly indicates the inability of general anesthesia to act as a thorough substitute for sleep. Contrary to what some sci-fi literature may convey, not all anesthesia can induce a sufficient night’s worth of sleep.
Conclusion
Although the effects of general anesthesia are not entirely understood at the molecular level, anesthesiology continues to evolve and innovate technologies that transform the field. Similar to recent advancements in artificial intelligence, smart devices, and self-driving cars, groundbreaking developments in nanotechnology are driving anesthesiology towards automation. Presently, an anesthetist provides general anesthesia by inducing the patient and maintaining anesthetic levels according to the patient’s blood pressure or heart rate. Prospective projects in nanotechnology propose an improvement to this process by creating brain-computer interfaces that will link electronic chips to the human nervous system. The chips, decorated with ion channels and a layer of cultured brain tissue, enable professionals to observe imaging of neuronal activity from an external computer. Professionals can determine the state of consciousness by reading the electrical signals and comparing them to expected data for the different phases of anesthesia. Anesthesiologists then deliver the appropriate dose according to the electrical reading in order to keep the patient fully sedated. Through developments such as this, the administration of anesthesia will be more accurate and less prone to mistakes. Ultimately, intraoperative awareness may be avoided [27].
However, the potential fruits of such developments do not erase the importance of the underlying mechanisms of general anesthesia. Without a sufficient understanding of the target sites, molecules, and signaling pathways involved, the proposed nanotechnology cannot accurately project electrical activity. More specifically, the electronic chips cannot be developed with the appropriate ion channels and placed in the right location for data acquisition. Until experts obtain a better sense of the neurophysiology responsible for general anesthesia and its postoperative effects, the topic of general anesthesia will remain a mystery.
References
- Sebel, P. S., Bowdle, T. A., Ghoneim, M. M., Rampil, I. J., Padilla, R. E., Gan, T. J., & Domino, K. B. (2004). The Incidence of Awareness During Anesthesia: a Multicenter United States Study. Anesthesia and Analgesia, 99(3), 833–839. https://doi.org/10.1213/01.ANE.0000130261.90896.6C
- Kent, C. D., Mashour, G. A., Metzger, N. A., Posner, K. L., & Domino, K. B. (2013). Psychological Impact of Unexpected Explicit Recall of Events Occurring During Surgery Performed Under Sedation, Regional Anaesthesia, and General Anaesthesia: Data from the Anesthesia Awareness Registry. British Journal of Anaesthesia, 110(3), 381–387. https://doi.org/10.1093/bja/aes386
- Mashour, G. A., Esaki, R. K., Tremper, K. K., Glick, D. B., O'Connor, M., & Avidan, M. S. (2010). A Novel Classification Instrument for Intraoperative Awareness Events. Anesthesia & Analgesia, 110(3), 813–815. https://doi.org/10.1213/ane.0b013e3181b6267d
- Cook, T. M., Andrade, J., Bogod, D. G., Hitchman, J. M., Jonker, W. R., Lucas, N., Mackay, J. H., Nimmo, A. F., O'Connor, K., O'Sullivan, E. P., Paul, R. G., Palmer, J. H., Plaat, F., Radcliffe, J. J., Sury, M. R., Torevell, H. E., Wang, M., Hainsworth, J., & Pandit, J. J. (2014). The 5th National Audit Project (NAP5) on Accidental Awareness During General Anaesthesia: Patient Experiences, Human Factors, Sedation, Consent and Medicolegal Issues. Association of Anaesthetists, 69(10), 1102–1116. https://doi.org/10.1111/anae.12827
- Bullard, T. L., Cobb, K., & Flynn, D. N. (2022). Intraoperative And Anesthesia Awareness. In StatPearls. StatPearls Publishing.
- Date, A., Bashir, K., Uddin, A., & Nigam, C. (2020). Differences Between Natural Sleep and the Anesthetic State. Future Science OA, FSO664. https://doi.org/10.2144/fsoa-2020-0149
- Moody, O. A., Zhang, E. R., Vincent, K. F., Kato, R., Melonakos, E. D., Nehs, C. J., & Solt, K. (2021). The Neural Circuits Underlying General Anesthesia and Sleep. Anesthesia and Analgesia, 132(5), 1254–1264. https://doi.org/10.1213/ANE.0000000000005361
- Brown, E. N. , Lydic, R. & Schiff, N. D. (2010). General Anesthesia, Sleep, and Coma. The New England Journal of Medicine, 363 (27), 2638-2650. DOI: 10.1056/NEJMra0808281.
- Steriade, M., Amzica, F., & Contreras, D. (1994). Cortical and Thalamic Cellular Correlates of Electroencephalographic Burst-Suppression. Electroencephalography and Clinical Neurophysiology, 90(1), 1–16. https://doi.org/10.1016/0013-4694(94)90108-2
- Mashour, G. A., Orser, B. A., Avidan, M. S., & Warner, D. S. (2011). Intraoperative Awareness. Anesthesiology, 114(5), 1218–1233. https://doi.org/10.1097/aln.0b013e31820fc9b6
- Diao, S., Ni, J., Liu, P., & Xia, W. (2014). Mechanisms of Action of General Anesthetics. Frontiers in Bioscience Landmark, 19(5), 747–757. https://doi.org/10.2741/4241
- Hantal, G., Fábián, B., Sega, M., Jójárt, B., & Jedlovszky, P. (2019). Effect of General Anesthetics on the Properties of Lipid Membranes of Various Compositions. Biochimica Et Biophysica Acta (BBA) - Biomembranes, 1861(3), 594–609. https://doi.org/10.1016/j.bbamem.2018.12.008
- X. Lever, M. J., Miller, K. W., Paton, W. D., & Smith, E. B. (1971). Pressure Reversal of Anaesthesia. Nature, 231(5302), 368–371. https://doi.org/10.1038/231368a0
- Cantor, R. S. (1997). Lateral Pressures in Cell Membranes: A Mechanism for Modulation of Protein Function. The Journal of Physical Chemistry B, 101(10), 1723–1725. https://doi.org/10.1021/jp963911x
- Franks, N. P., & Lieb, W. R. (1984). Do General Anaesthetics Act by Competitive Binding to Specific Receptors? Nature, 310(5978), 599–601. https://doi.org/10.1038/310599a0
- Gottesmann C. (2002). GABA Mechanisms and Sleep. Neuroscience, 111(2), 231–239. https://doi.org/10.1016/s0306-4522(02)00034-9
- Zimmerman, S. A., Jones, M. V., & Harrison, N. L. (1994). Potentiation of Gamma-Aminobutyric AcidA Receptor Cl- Current Correlates with in vivo Anesthetic Potency. The Journal of Pharmacology and Experimental Therapeutics, 270(3), 987–991.
- Franks N. P. (2008). General Anaesthesia: From Molecular Targets to Neuronal Pathways of Sleep and Arousal. Nature Reviews. Neuroscience, 9(5), 370–386. https://doi.org/10.1038/nrn2372
- Zielinski, M. R., McKenna, J. T., & McCarley, R. W. (2016). Functions and Mechanisms of Sleep. AIMS neuroscience, 3(1), 67–104. https://doi.org/10.3934/Neuroscience.2016.1.67
- Sonner, J. M., Antognini, J. F., Dutton, R. C., Flood, P., Gray, A. T., Harris, R. A., Homanics, G. E., Kendig, J., Orser, B., Raines, D. E., Trudell, J., Vissel, B., & Eger, E. (2003). Inhaled Anesthetics and Immobility: Mechanisms, Mysteries, and Minimum Alveolar Anesthetic Concentration. Anesthesia & Analgesia, 97(3), 718–740. https://doi.org/10.1213/01.ane.0000081063.76651.33
- Patel, A. J., Honoré, E., Lesage, F., Fink, M., Romey, G., & Lazdunski, M. (1999). Inhalational Anesthetics Activate Two-Pore-Domain Background K+ Channels. Nature Neuroscience, 2(5), 422–426. https://doi.org/10.1038/8084
- Hammond, R., Christie, M., & Nicholson, A. (2008). Opioid Analgesics. ScienceDirect, 309–329. https://doi.org/10.1016/b978-070202858-8.50016-6
- Kameyama, A., Asai, H., Nomoto, M., Ohno, S., Ghandour, K., Ohkawa, N., Saitoh, Y., Yamazaki, M., & Inokuchi, K. (2022). Sevoflurane-Induced Amnesia is Associated with Inhibition of Hippocampal Cell Ensemble Activity After Learning. Biology Open, 11(12). https://doi.org/10.1242/bio.059666
- Leslie, K., Skrzypek, H., Paech, M. J., Kurowski, I., & Whybrow, T. (2007). Dreaming During Anesthesia and Anesthetic Depth in Elective Surgery Patients: a Prospective Cohort Study. Anesthesiology, 106(1), 33–42. https://doi.org/10.1097/00000542-200701000-00010
- Leslie, K., & Skrzypek, H. (2007). Dreaming During Anaesthesia in Adult Patients. ScienceDirect, 21(3), 403–414. https://doi.org/10.1016/j.bpa.2007.05.003
- Tung, A., Bergmann, B. M., Herrera, S., Cao, D., & Mendelson, W. B. (2004). Recovery From Sleep Deprivation Occurs During Propofol Anesthesia. Anesthesiology, 100(6), 1419–1426. https://doi.org/10.1097/00000542-200406000-00014
- Agarwal, A. (2012). The Future of Anaesthesiology. Indian Journal of Anaesthesia, 56(6), 524–528. https://doi.org/10.4103/0019-5049.104567