Think back to the most memorable place you’ve been. Whether it be the alleys and roads that bisect to make up your childhood neighborhood or a distant country you visited for your first vacation, visualize the scene in your mind. I see a cherry tree with bright red cherries dangling from the branches. The tree stands in the middle of a backyard, and I am placed between the tree and my house. To the left is a cul-de-sac, one I cannot entirely remember, and to my right a faded brown fence. This was my first home. The scenery is not much: a tree, a fence, and a circular arrangement of houses, yet the collective experiences that took place in the backyard are engrained in my memory. This teeming vessel, overflowing with beautiful reenactments of time and space, is a product of incredibly complex and interconnected systems that can be attributed to the hippocampus. Although research is far from complete, navigation and memory seem to be very closely tied to hippocampal activities. Our neurons fire in specific locations to determine position, and each position is stored as a map within the brain. These maps are ever-changing, constantly rotating and scaling up or down depending on where you are. This phenomenon is responsible for finding your way home at night, remembering where you put your phone, and even recalling which food truck has your favorite food. Exploring research in several fields will help explain the mystery of mental maps.
The brain uses specialized neurons that detect direction and movement to create mental maps. One of those neurons is a place cell. Place cells are located in the hippocampus and fire when you are in a certain area [1] . This area is called a firing field and is specific to each place cell. However, place cells can form networks between themselves, which create a larger representation of an area. This representation can be remapped at a network level, meaning one tangle of place cells can code for your bedroom and your kitchen, depending on where you are standing.
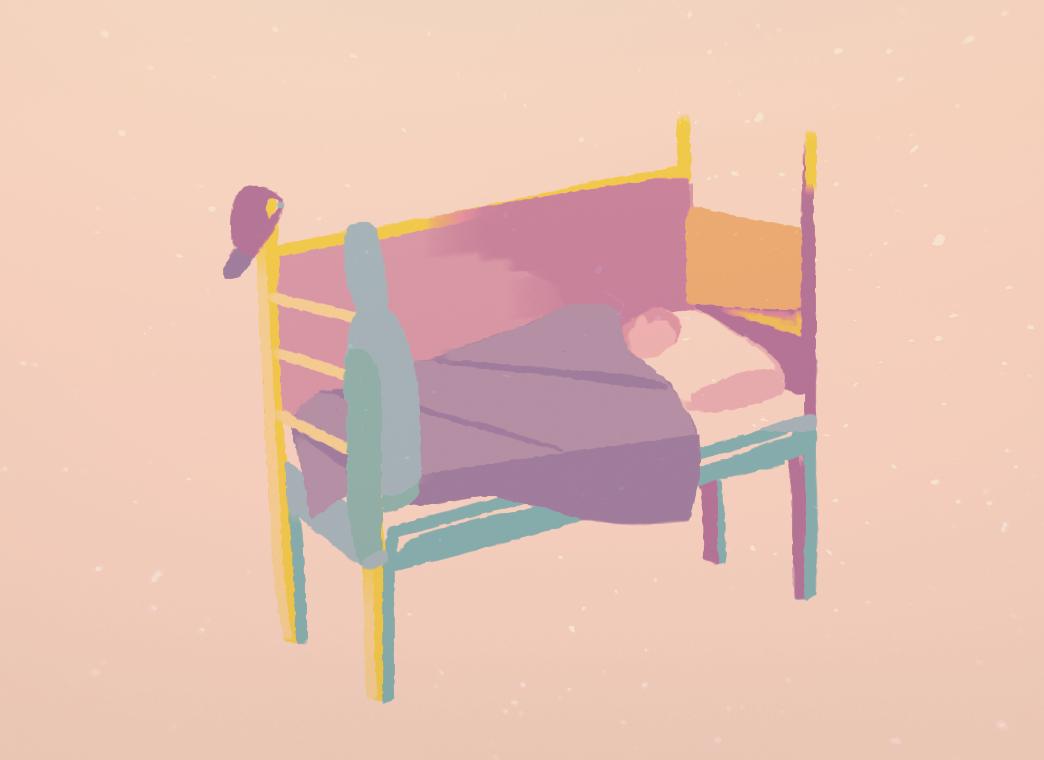
The first step in establishing a focal point for a map is one’s place. A place is a location that is not marked by any recognizable cues and thus is only recognizable when related to other landmarks [1] . Imagine standing in a park where all you can see is grass, but you do not know where you are—that is one place. If you were to see a water fountain you recognized, you would be able to tell which park you were in and possibly even your distance relative to local landmarks. Although it feels simple, the ability to recognize your own location as well as how you relate to your environment is an incredibly complex task.
The formation of a mental map begins with a two-part location-determining module. The first of these systems is the allocentric system. The allocentric system establishes the position of an arbitrary point by its distance between several landmarked positions [1] . For example, allocentric navigation would determine the distance of a patch of grass between two food trucks. The second system is the path integration system, or egocentric system. This system determines location relative to a starting point, such as your home or front door [1] . So, if you were to leave home to get a burrito from one of the food trucks mentioned earlier, you would be able to estimate the distance from your home to the truck. Then, with the information from the allocentric system, you could determine the distance it would take to walk from the food truck to the patch of grass to enjoy a delicious picnic. The path integration system does this by recording information from receptors in the limbs about the distance travelled and changes in direction [1] . Think of this as a biological Fitbit that tracks your distance travelled by the strides you take.
Research on the area responsible for these systems concludes that the cells involved in navigation most likely reside in the hippocampus due to the heavy use of memory in forming mental maps. Studies conducted by Morris et al. revealed some interesting results about the nature of mental maps [2] . Mice that took part in the Morris water maze began by finding a hidden platform beneath a pool of water and memorized the location of that platform through repetition. To test the correlation between hippocampal neurons and navigation, researchers lesioned the hippocampi of mice and recorded the time it took for them to complete the maze. These mice took significantly longer to find the platform than control mice, thus exhibiting a loss of orientation [2] . However, in an experiment to determine spatial learning, the platform was made visible to the mice in one trial. After 30 seconds, the platform was hidden and the mice were tested again. In the first trial, both lesioned and control mice took the same amount of time to escape the maze. Yet in the second trial, lesioned mice took a considerably longer time escaping the maze compared to the control mice [2] . The inability of the lesioned mice to remember the location of the platform is attributed to damage in the hippocampus, which indicates the role of hippocampal cells in spatial learning. These results show that the hippocampus is crucial for navigation and memory.
Now that the hippocampus has been established as the center of navigation, a deeper investigation should be conducted to determine which neurons are responsible for this. Place cells are the primary candidates for involvement in navigation; however, evidence is required to solidify this claim. Lavilleon et al. experimented with an artificial place-reward system to observe whether a place cell that was rewarded for signaling would drive an animal to its associated location [3] . By placing electrodes that were known to induce reward onto place cells in mice, Lavilleon could test whether the mice would enter that place field more often than the controls. It turns out that the time spent in the associated place field increased four to five-fold for mice with the electrode implants. At least for electrode-bearing mice, place cells and navigation do in fact go hand in hand [3] .
The process by which mental maps are created in the brain can be quite complex, despite neurons having the simplified ability to communicate in binary mechanics: yes or no, 0 or 1. Given the complex nature of the information being sent between neurons, these cells require methods of encoding that allow them to convey a multitude of information in a single yes or no. Neural modulation is the principal that a neuron can be altered to affect its firing abilities or what it is firing for. One form of neural modulation is firing rates. By differentiating their firing rates, neurons can communicate on a level independent of their binary mode of signaling. This mechanism is important in mental representations because place cells need to relay an enormous amount of information while simultaneously integrating multiple areas of the body and brain to build a mental map. Information of this magnitude cannot be sent in 1s and 0s alone, so differing firing rates can be used to associate a condition that needs to be met for a particular neuron to fire at its maximum frequency [4] .
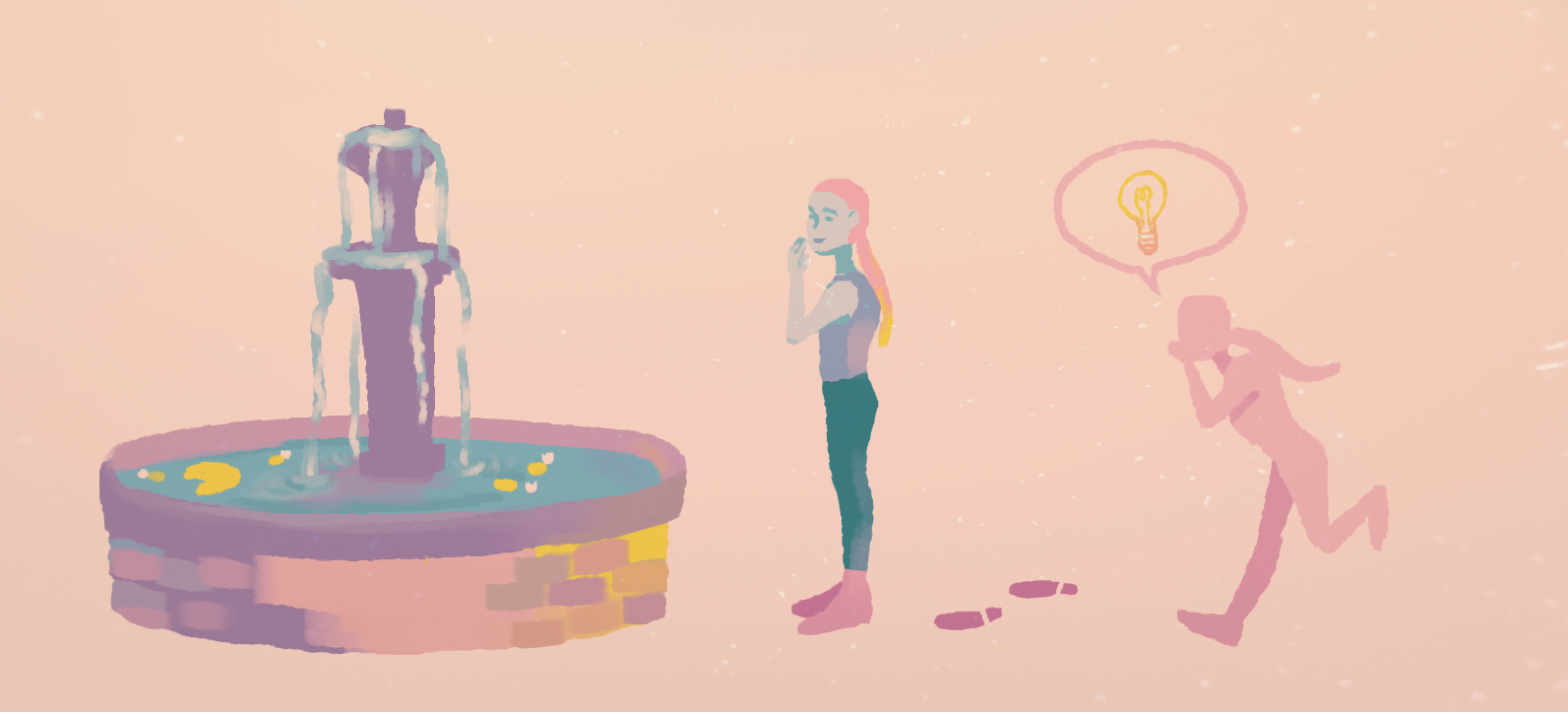
Along with firing rate, neural modulation also involves spike timing. This is the specific amount of time after a stimulus that a neuron will fire. One type of spike timing is the theta wave: a neural oscillatory pattern that creates a theta rhythm, or a pattern of neuronal firing that resembles a theta curve [5] . Lesion studies performed on the medial septum and fornix reduced the hippocampal theta rhythm, which was followed by a decrease in memory-guided tasks [5] . Moreover, studies on theta rhythms in rats during locomotion depict how memory is used in navigation. Vertes et al. theorized that theta rhythm is used as a short-term memory tag [6] . When rats move around their environment, theta waves are present and can be recorded. One hypothesis for how memory is stored claims that one signal opens NMDA channels, then another signal enacts functional change that is a memory. Vertes viewed theta rhythm as the opening signal that allows animals to create a new memory [6] . In this regard, theta waves could be used to initiate the construction of or addition to a mental map by facilitating memory creation.
Another type of neural modulation is through chemical changes. To test how amphetamine affects spatial memory, Packard et al. injected the hippocampi of mice with d-amphetamine and had them go through a Morris water maze [7] . Compared to a control group of normal mice, the d-amphetamine mice took significantly less time to complete the maze. These results indicate how the modulation of neurons can lead to stronger memory consolidation [7] . Whether this modulation occurs in only a portion of the brain or several was a key question that was addressed by Packard’s research. By injecting lidocaine, an anesthetic that blocks neural activity, into the amygdalas of half of the mice, Packard was able to determine whether modulation occurred exclusively in the amygdala or if it spread to other regions of the brain [7] . Mice with lidocaine and d-amphetamine performed similarly in a Morris water maze to mice that only had d-amphetamine injected [7] . It seems as though neural modulation occurs within the entire brain, or at least in circuits that run through several areas, and is not centered in the amygdala. So if changes in neural behavior lead to better memory, how exactly does it work?
According to Grill-Spector et al., the model that explains repetitive suppression that is most intuitive for memory is the facilitation model [8] . This theory states that neural firing times are decreased when responding to a stimulus a second time. Effectively, facilitation causes an increase in neural potentiation that leads to the faster recognition of stimuli. In other words, when a stimulus is seen a second time, the neurons that are responsible for recognizing that object can communicate at twice the normal speed; thus, the signal is processed faster, which means less time staring at an object trying to remember what it is, whether you like it or not, and what to do with it. It is theorized that repetition in the facilitation model leads to an increase in correct predictions of that object [8] . Navigation-wise, this demonstrates how a mental map can become stronger or more detailed with repetition. James et al. explained this phenomenon by differentiating diagnostic neurons and non-diagnostic neurons in the memory formation process [9] . James represented an object as a group of neurons in the temporal lobe, with neurons that encoded features necessary for recognition as diagnostic and others as non-diagnostic. Repeated exposure to the same object led to a decrease in activity in non-diagnostic neurons while the diagnostic ones were untouched. After a while, non-diagnostic neurons stopped firing completely. At this point, the diagnostic neurons underwent sharpening, which is an increase in their efficiency at signaling important features. In terms of peak signaling, sharpening led to a shift in time of peak activity, which means recognition occurred at a faster rate [9] . The facilitation and sharpening models of memory formation and retrieval explain how one can recognize a familiar place faster and more accurately than an unfamiliar one. Faster recollection is tied to better navigation through quicker identification of objects. These processes that result in better memory consolidation are a product of neural modulation, whether it be changes in firing rates or chemical imbalances. Dynamic brain regulation seems to be an important aspect of the formation of mental maps.
The dynamic nature of orientation begs the question of whether or not mental maps change with time. To test this theory, Rolls et al. had monkeys watch a monitor that displayed a variety of pictures that would either change location on the screen or would stay in the same place [10] . This experiment was meant to test hippocampal neurons and their reaction to novel stimuli such as a new photograph, a new location, or a combination of the two. It was found that some neurons fired when a picture was shown for the first time, while other neurons fired when that picture was in a certain location. However, all cells fired with less intensity when an identical picture was shown a second time [10] . Although memory is a difficult topic to define neurologically, some aspects of its behavior are well known. One such aspect is repetitive suppression. Scientists believe that repetitive suppression is the suppression of a neuron’s firing rate when it has signaled for the same stimulus a second time, which would account for the depressed firing rate the monkeys’ neurons experienced.
The beauty of mental maps is their limitless versatility. We can form mental maps in any environment with just a glance at our surroundings. In order to determine how mental maps rely on memory, Hafting et al. tested the place cells of rats in areas exposed to light and recorded how they changed when those areas were completely dark [11] . They found that the spacing of the place cells,
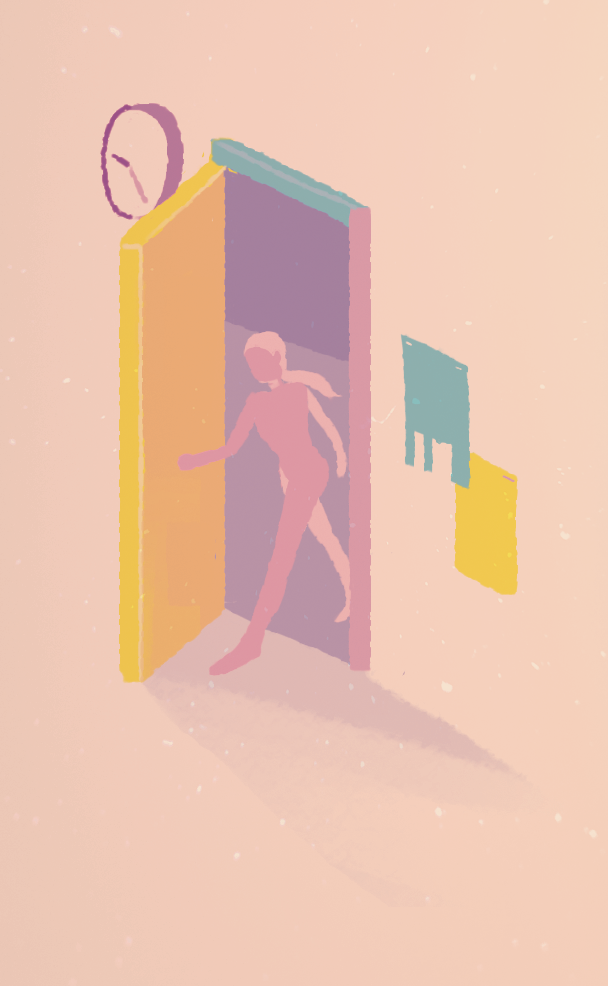
the average firing rate, and the spatial information per spike were not affected by darkness. There was a slight shift that researchers theorized to be due to a lack of allocentric cues, or cues that help determine your position relative to other objects [11] . What is interesting is that while the “outlined” map was intact, there was a weak displacement in spatial correlation [11] . On a macroscopic level, this is the equivalent of someone recreating a living room. They have all the right furniture as the original, but the entire room is shifted 30 degrees. These findings point to the role of memory in storing a grid that relates objects to one another by distance and our ability to recreate that grid from memory, as indicated by the rats’ grid being present and relatively unchanged even in darkness.
To understand the principal behavior behind orientation, Young et al. tested rats as they went through a maze. Young discovered that there were three complex-spike cell types in the hippocampus, and each had an effect on mental representation [12] . The first of these cells were cue cells, which fired when the rat stepped over a certain pattern on the ground, regardless of where that pattern was. The second type of cells were place cells, cells that fired only when the rat was in a specific location. Finally, there were cells that fired only when the rat was in a specific location relative to other landmarks; for example, if it was a certain distance away from the start, regardless of the direction [12] . The combination of these cell types explains how the brain can map an area so quickly and with such precision. These data show that many features can be stored in the hippocampus, in addition to space; thus, spatial representation may not be the sole function of the hippocampus. The interaction between these cell types is highlighted in Wiener et al.’s research. Wiener had rats sample odors at different locations and recorded the firing rate of these cells [13] . Roughly half of the cue cells increased their firing rates when the rat smelled a certain odor, when it was in a specific area of the box, or a combination of the two [13] . It is possible that all the characteristics of a location, such as scents and relative and absolute distance, can be compiled to form a more complete representation of an area. The brain’s ability to accomplish this is a phenomenal feat that cannot be rivaled by even the most advanced computer. The level of sophistication place cells possess when constructing mental maps should be considered a testament to their unbelievable specificity. Being able to differentiate between a wide range of information and consolidating that information into the representation of an area, while also taking into account subtle nuances of the environment, is a significant task.
“Mental map” is such a simple term. It does not evoke a feeling of confusion or debate, although perhaps it should. The formation, utilization, and rearrangement of mental maps are, as has already been stated, incredibly complex. Tens of systems, with hundreds of jobs and thousands of connections compromised of millions of cells all work in perfect harmony to create a product most people undervalue. With the newfound understanding of mental maps you have recently gained, think again to the most memorable place you have been. Picture yourself in the environment like a pin on a map. Take notice of the landmarks around you and imagine their distances relative to your own. What about any interesting features or cues, such as smells, vivid memories within the mental map, or expectations of joy or pain associated with the map? Think of the intricate systems and mechanisms working inside your brain to create the scene you are now imagining. Behind the curtain is a plethora of modulation, plasticity, and specialization that goes unnoticed. However, hopefully now, with new knowledge, you can appreciate the inner workings of mental maps.
- Bures, J. (1997). Place cells and place navigation. Proceedings Of The National Academy Of Sciences Of The United States Of America, 94(1), 343-350. Retrieved from http://www.pnas.org/content/94/1/343.full
- Morris, R., Garrud, P., Rawlins, J., & O’Keefe, J. (1982). Place navigation impaired in rats with hippocampal lesions. Nature, 297(5868), 681-683. http://dx.doi.org/10.1038/297681a0
- de Lavilléon, G., Lacroix, M., Rondi-Reig, L., & Benchenane, K. (2015). Explicit memory creation during sleep demonstrates a causal role of place cells in navigation. Nature Neuroscience, 18(4), 493-495. http://dx.doi.org/10.1038/nn.3970
- Mizuseki, K., & Buzsáki, G. (2013). Preconfigured, Skewed Distribution of Firing Rates in the Hippocampus and Entorhinal Cortex. Cell Reports, 4(5), 1010-1021. http://dx.doi.org/10.1016/j.celrep.2013.07.039
- Hasselmo, M. (2005). What is the function of hippocampal theta rhythm?—Linking behavioral data to phasic properties of field potential and unit recording data. Hippocampus, 15(7), 936-949. http://dx.doi.org/10.1002/hipo.20116
- Vertes, R. (2005). Hippocampal theta rhythm: A tag for short-term memory. Hippocampus, 15(7), 923-935. http://dx.doi.org/10.1002/hipo.20118
- Packard, M., Cahill, L., & McGaugh, J. (1994). Amygdala modulation of hippocampal-dependent and caudate nucleus-dependent memory processes. Proceedings Of The National Academy Of Sciences, 91(18), 8477-8481. http://dx.doi.org/10.1073/pnas.91.18.8477
- Grill-Spector, K., Henson, R., & Martin, A. (2006). Repetition and the brain: neural models of stimulus-specific effects. Trends In Cognitive Sciences, 10(1), 14-23. http://dx.doi.org/10.1016/j.tics.2005.11.006
- James, T., & Gauthier, I. (2005). Repetition-induced changes in BOLD response reflect accumulation of neural activity. Human Brain Mapping, 27(1), 37-46. http://dx.doi.org/10.1002/hbm.20165
- Cahusac, P., Miyashita, Y., & Rolls, E. (1989). Responses of hippocampal formation neurons in the monkey related to delayed spatial response and object-place memory tasks. Behavioural Brain Research, 33(3), 229-240. http://dx.doi.org/10.1016/s0166-4328(89)80118-4
- Hafting, T., Fyhn, M., Molden, S., Moser, M., & Moser, E. (2005). Microstructure of a spatial map in the entorhinal cortex. Nature, 436(7052), 801-806. http://dx.doi.org/10.1038/nature03721
- Young, B., & Fox, G. (1994). Correlates of Hippocampal Complex-Spike Cell Activity in Rats Performing a Nonspatial Radial Maze Task. Journal Of Neuroscience, 14(11).
- Wiener, S., Paul, C., & Eichenbaum, H. (1989). Spatial and behavioral correlates of hippocampal neuronal activity. Journal Of Neuroscience, 9(8), 2737-2763.