Within the human body, many conditions need to be kept within a physiologically optimal range for the system to function properly. This is highly important at the cellular and subcellular level, where minute deviations in temperature, acidity, or nutrient availability can have drastic consequences, which manifest macroscopically as health problems. Accordingly, the concept of maintaining a stable internal condition in an organism, called homeostasis, is prevalent throughout biology.
This principle is aptly displayed in the body’s maintenance of energy homeostasis through regulation of energy intake and expenditure. Since animals get energy from the food they eat, regulation of energy intake in animals occurs through adjustment of the animal’s patterns of food intake. These patterns of food intake, referred to as feeding behavior, are regulated by homeostatic neural circuits that respond to molecular signals indicating how much energy the organism has and how much it needs in both acute and long-term timescales. However, feeding behavior is more complicated than just energy balance because reward systems also play a role. Neural circuits mediating reward can cause various feeding behaviors to be pleasurable or unpleasurable, and this can also promote feeding behavior that aligns with the organism’s energy requirements. Disruption of these systems mediating energy homeostasis and food reward can result in abnormal feeding behavior, including both overeating and undereating, and can have severe health consequences. As such, studying the homeostatic and hedonic neural circuits underlying feeding behavior can provide valuable insight into principles governing energy homeostasis as well as the issues underlying abnormal feeding behavior in cases of disease.
Regulation of Feeding Behavior by the Energy Homeostasis System
Think of the thermostat in your home. In order for it to regulate the temperature, it must have both the ability to detect the temperature at any given time and the capacity to correct it when it goes out of range. Likewise, homeostatic systems must also have the ability to sense and correct the condition they regulate in order to keep it within a physiologically optimal range. In 1953, British scientist Gordon C. Kennedy put forth a model of energy homeostasis regulation called the lipostatic model, in which energy sensing and correcting are mediated by the hypothalamus, a brain structure that orchestrates homeostasis throughout the body. In the model, Kennedy proposed that the hypothalamus senses chemical signals circulating in the bloodstream in proportion to the amount of body fat and adjusts feeding behavior accordingly [1][2]. Over six decades later, our current understanding of energy homeostasis fits within the framework of this model.
Some of the first studies to determine the key molecular signals in energy homeostasis were those done on the mouse genes obese (ob) and diabetes (db), each respectively named after the effect of mutations in those genes. Much of what is known now about these genes comes from a series of elegant experiments conducted in the 1970s by Douglas L. Coleman and colleagues. These experiments employed a technique called parabiosis in which the circulatory systems of two live animals are surgically joined together so the two individuals share all the same molecular signals in their bloodstreams [2]. Using this approach, Coleman demonstrated that the ob gene encoded a peptide called leptin, which suppresses food intake, and db encoded the leptin receptor [3]. Later studies in mice showed leptin was directly secreted from fat cells, called adipocytes, into the bloodstream, and that leptin secretion was decreased in fasted mice [4]. Leptin is generally thought of as an adiposity-related signal because the amount of leptin in the bloodstream in any given individual is proportional to the individual’s body fat, and it suppresses feeding behavior [5]. In addition to leptin, there are a number of other molecular signals that also serve to suppress feeding behavior, either in proportion to body fat-like leptin or in a more short-term manner related instead to the contents of the stomach [6-10]. The cumulative effect of leptin and these other molecular signals is to decrease an animal’s food intake when it has sufficient energy, either from a recent meal or from energy stored as fat.
Conversely, molecular signaling also plays an important role in inducing hunger under conditions of energy deficiency. The primary peripheral hunger signaling molecule is ghrelin, a peptide secreted from the stomach. The concentration of ghrelin in the bloodstream is increased following food deprivation, and direct infusion of ghrelin into the brain causes increased food intake [11]. Thus, ghrelin is the functional opposite of leptin, increasing an animal’s food intake when it is energy deficient.
These hormones influence feeding behavior by acting on several distinct areas, or nuclei, within the hypothalamus. Although as many as five distinct hypothalamic nuclei have been shown to be essential for regulating feeding behavior, one particularly instructive model of energy homeostasis regulation focuses on a single hypothalamic nucleus, the arcuate nucleus of the hypothalamus (ARC) [2]. The ARC is positioned near capillaries that run close to the hypothalamus, exposing it to molecular signals in the bloodstream. Two distinct subpopulations of neurons are present within the ARC: those that express the peptide proopiomelanocortin (POMC neurons) and those that co-express the peptides agouti-related protein and neuropeptide-Y (AgRP/NPY neurons) [2]. Experiments involving genetically engineered light- or drug-dependent activation of subsets of neurons (referred to as optogenetics and chemogenetics, respectively) have shown that activation of AgRP/NPY neurons in mice causes impressive increases in food intake [12][13]. POMC neurons, by contrast, suppress feeding when activated [2].
How is this system influenced by satiety signaling from leptin and hunger signaling from ghrelin? Leptin signaling suppresses feeding by inhibiting AgRP/NPY neurons and activating POMC neurons, whereas ghrelin signaling promotes feeding by activating AgRP/NPY neurons and inhibiting POMC neurons [2][5]. This model is an oversimplification of the neural circuitry and molecular signaling underlying feeding behavior, given that many other nuclei and molecular signals are involved, including ones that directly interact with these neurons in the ARC but are not mentioned here. However, the model is still instructive because it illustrates the principles underlying neural regulation of energy homeostasis. That is, peripheral molecular signals, which reflect physiological energy levels, directly interact with structures regulating feeding behavior, such that energy deficiency or sufficiency is reflected in the organism’s food intake.
The Role of Reward Neural Circuitry in Shaping Feeding Behavior
Direct control of food intake by energy homeostasis neural circuitry makes practical sense, but you probably know from experience and observation that decisions about food intake are more complicated than just energy homeostasis. For example, both humans and animals will at times eat foods that are rewarding due to their palatability even in the absence of hunger or need. This tendency can be explained by the fact that feeding behavior is not only shaped by neural circuits mediating energy homeostasis but also by neural circuits mediating pleasure and reward, referred to as hedonic circuits. These reward processing systems include a neural circuit called the mesolimbic pathway as well as structures in the hypothalamus.
The mesolimbic pathway is a dopaminergic projection from the ventral tegmental area (VTA) to the nucleus accumbens (NAc). This pathway is associated with motivation and pleasure, and disordered activity in this pathway has been strongly implicated as a physiological feature of drug addiction [14]. Upon first exposure to a food reward, signaling by dopaminergic neurons in the VTA increases in frequency, resulting in increased dopamine release. During subsequent exposures to the food reward, stimuli associated with the food (e.g. scent) could activate this same reward pathway [15]. Dopamine signaling in this neural circuit indicates motivation and sensation of pleasure, so activation of the circuit even solely by food-associated stimuli demonstrates the potent appeal of the act of feeding. VTA neurons express leptin receptors, and the binding of leptin to VTA neurons results in decreased VTA neuronal activity and decreased food intake [16]. This result can be interpreted as leptin causing a decrease in how rewarding food is, which could reinforce decreased food intake. Ghrelin, the hunger hormone, also has a role in influencing hedonic neural circuitry, demonstrated by the fact that multiple structures in the mesolimbic system have ghrelin receptors. The activity of ghrelin in the VTA has been shown to be essential for establishing the rewarding nature of palatable food and thus may play a role in encoding food reward [17].
Within the hypothalamus, a region called the lateral hypothalamic area (LHA) has been known to be involved in feeding behavior since the 1950s, when researchers found that rats with lesions in this area exhibited decreased food intake [18]. Further investigation in 1962 showed that rats with LHA lesions had a different hedonic encoding of food: when presented with palatable, sweetened milk, these rats would exhibit an aversive reaction, including a gaping mouth expression that is associated with aversion [19]. Much later, in 1998, researchers found that a subset of neurons in the LHA express a peptide called orexin, which causes increased food intake when directly infused into the brain [20]. These LHA orexin neurons are also active during reward-seeking behavior [21][22]. Indeed, connections between LHA orexin neurons and the NAc as well as the VTA have been shown to be essential for one commonly used experimental model of food preference [23]. Orexin-expressing neurons are just one subpopulation of neurons among many in the LHA that come together to influence both feeding behavior and food reward processing in complex ways [24]. Interestingly, some neurons in the LHA also express the leptin receptor, and mice deficient in this LHA leptin receptor exhibit early-onset obesity, providing another possible explanation for the altered feeding behavior observed in LHA-lesioned mice [25]. However, the role of leptin signaling in the LHA is unclear in light of conflicting results and numerous proposed mechanistic explanations [26][27][28].
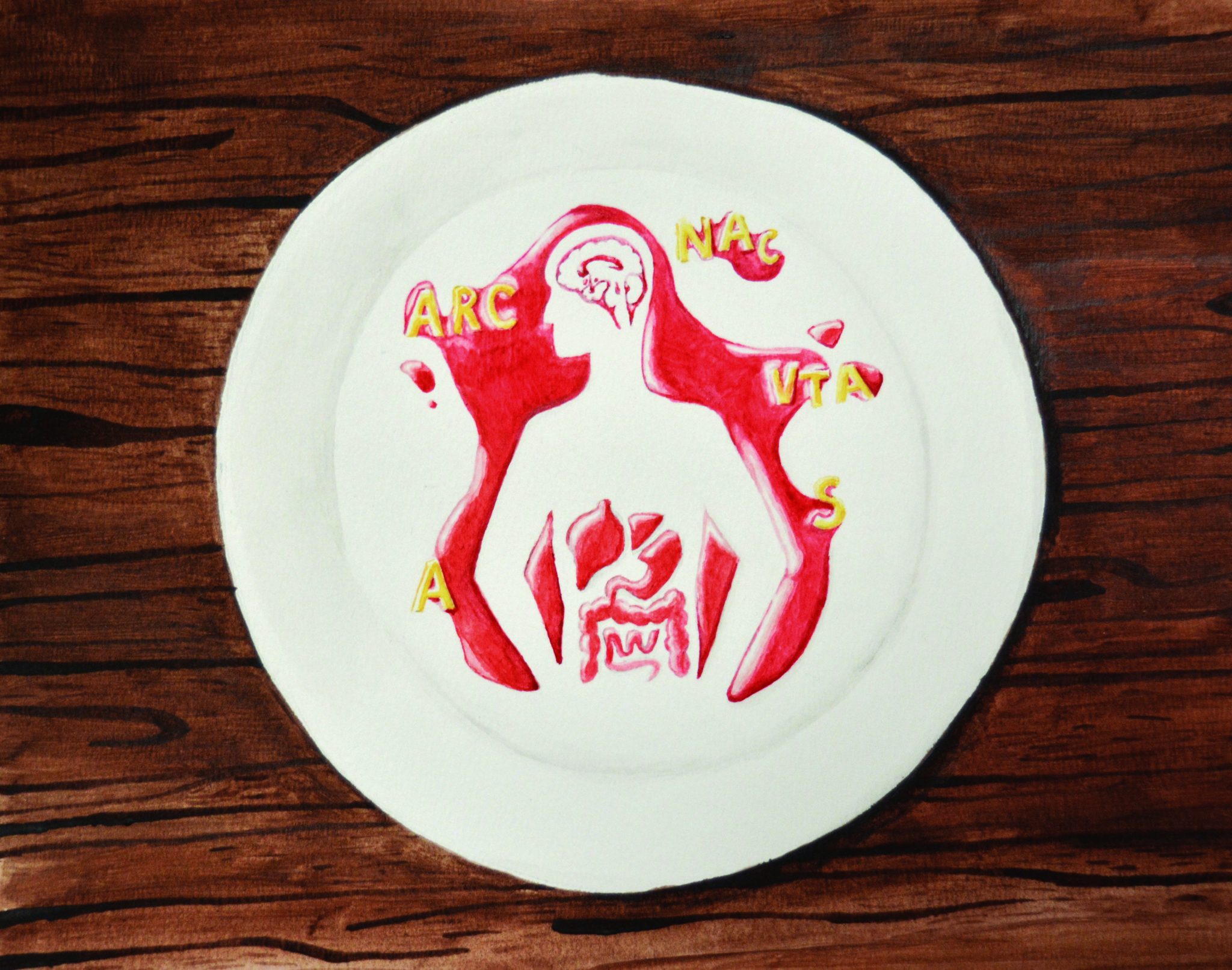
As such, feeding behavior is shaped not only by neural circuits that mediate homeostasis but also by brain centers and circuits that mediate reward. The mesolimbic system is involved in motivation and pleasure associations with food, and signaling by leptin and ghrelin in these neurons further promotes homeostatic feeding behavior in response to satiety signaling and food reward. The LHA has an important role in food reward processing that arises from the activity of several subpopulations of neurons within the LHA. Taken together, these effects illustrate a role for hedonic neural circuitry in shaping feeding behavior by providing motivation for food intake and by establishing food preference and food reward.
Homeostatic and Hedonic Neural Circuitry in Abnormal Feeding Behavior
Even with both homeostatic and hedonic circuits shaping feeding behavior to align with the energy needs of an animal, it is possible for food intake to sometimes be non-homeostatic—that is, to conflict with the energy needs of the organism. Perhaps the most prevalent form of non-homeostatic feeding behavior is overeating, which lies at the heart of the current national obesity epidemic. Indeed, epidemic is a fitting term, given that the prevalence of obesity in the United States was 39.8% in adults and 18.5% in youth in 2015-2016, according to a report by the National Center for Health Statistics [29]. From the standpoint of energy homeostasis and reward neural circuitry, overeating can be compellingly modeled as a form of addiction. The psychological patterns of motivation underlying chronic overeating strongly parallel those underlying drug abuse, giving rise to the scientific community’s shift toward seeing food addiction as a legitimate condition, although controversy over the model still exists [30]. One parallel between drug abuse and food addiction is the development of tolerance, or decreased response to a substance or stimulus over repeated use or exposure [31]. Diet-induced obesity results in leptin resistance in both the ARC and the VTA, among other structures, and thus causes satiety signaling to have a lowered effect in both the homeostatic and hedonic circuits modulating feeding behavior [32]. In other words, chronic overeating can result in resistance to satiety signaling, which would further promote overeating.
On the other hand, undereating is less widespread but can very quickly have serious negative health impacts. Loss of appetite for food, referred to as anorexia, often coincides with other conditions of disease or distress. A wide range of abnormal conditions and diseased states can be accompanied by anorexic behavior—ranging from environmental stress to cancer—and different causes have been proposed for these behaviors under different circumstances [5, 33-35]. One emerging notion in research of stress- and cancer-induced anorexia worth highlighting is the role of a nucleus in the brainstem called the parabrachial nucleus (PBN) [5]. In addition to purportedly encoding satiety in general, neurons in the PBN are activated by various kinds of aversive stimuli—ranging from the feeling of sickness associated with cancer to the feeling of pain—and strongly suppress feeding behavior when active [36-38]. The multiplicity of aversive stimuli that activate this emergency food intake-suppressing circuit suggest a possible mechanism for stress-induced anorexia, another form of loss of appetite brought on by distressing stimuli.
The severe health impacts of the disruptions of the normal homeostatic and hedonic regulation of food intake seen in these diseases are a testament to the intricacy of the regulatory system itself. The molecular and neuronal signaling in the system can respond effectively under normal conditions to keep food intake at an appropriate level; however, the system can be broken by too much signaling or by disease conditions that interfere with molecular signals or neural circuits.
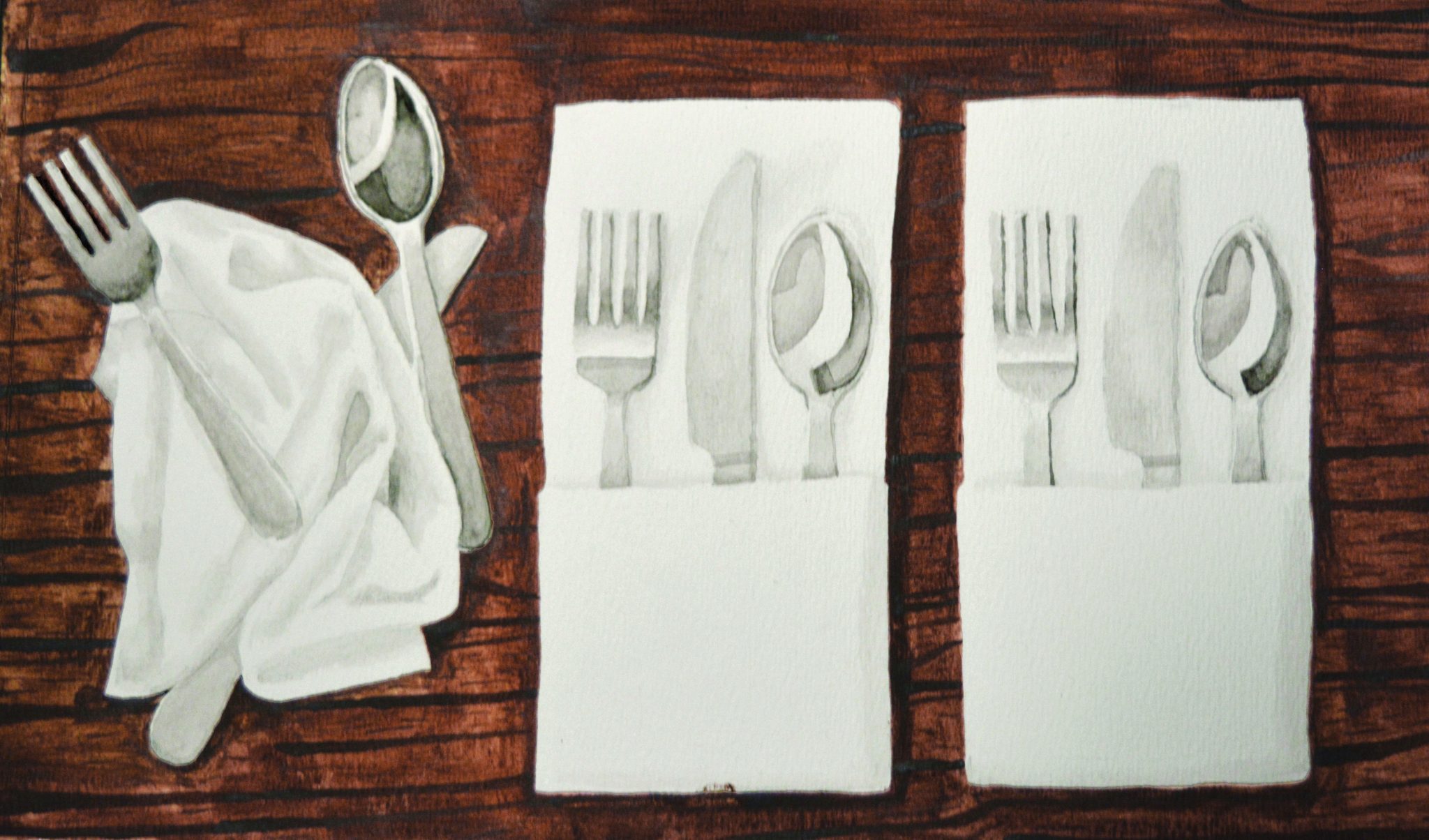
Looking Forward: Applications of Feeding Behavior Research
Over the last 70 years, our understanding of the neural basis of feeding behavior has progressed from conceptual models and knowledge of what brain structures might be involved to a detailed understanding of the neural circuitry and molecular signaling patterns that shape feeding behavior. These advances have allowed for a greatly increased understanding of the regulation of normal feeding behavior and also for application of that understanding to the investigation of diseases. This in turn paves the way for developing targeted treatments for diseases characterized by non-homeostatic eating. Much of the research on energy homeostasis has been motivated by interest in better understanding and being able to treat obesity, an ailment for which there are currently only modestly effective pharmacological or behavioral treatment strategies, despite its high prevalence. The first therapeutic approach, healthier eating and exercise patterns, often fails to produce substantial, lasting improvement [39]. Finding an effective pharmacological treatment has also been challenging due to the severe side effects of most candidate obesity drugs [39]. Bariatric surgery, applied in very severe cases of obesity, has appreciable costs and risks, and patients often regain weight after the surgery [39]. As the scientific community has elucidated the signaling underlying energy homeostasis, more effective drugs have been developed targeting structures in the brain that regulate feeding behavior, making the treatment outlook more promising.
In addition to these pharmacological approaches, one interesting new treatment strategy has emerged within the last decade: direct electrical stimulation of relevant structures to modify their activity [40]. One such treatment targeting the vagus nerve, which carries signals that are eventually relayed to the food intake-suppressing PBN, has already been approved by the Food and Drug Administration (FDA) and was shown to cause sustained, significant weight loss over a two-year period [41]. Other treatments targeting brain structures for stimulation, for instance an approach called deep brain stimulation typically used to treat movement disorders, are currently being designed and tested. [42] One such treatment targeting the LHA has already undergone an FDA-approved pilot study and showed modest effectiveness, and a number of other structures have been proposed as potential deep brain stimulation targets, including the NAc [42][43].
These novel, research-based therapeutic approaches indicate a promising future for the treatment of obesity, and it is through understanding of the neural circuitry and molecular signaling involved in shaping feeding behavior that these treatments have been conceptualized and developed. In addition to paving the way for development of new treatments, research on the systems regulating feeding behavior has revealed the complex interplay between homeostatic and hedonic systems in shaping behavior. As such, continued research on the homeostatic and hedonic basis for feeding behavior will yield valuable insight into what shapes patterns of food intake in normal and abnormal conditions.
References
- Kennedy, G. C. (1953) The role of depot fat in the hypothalamic control of food intake in the rat. Proc. R. Soc. Lond. B 1953 140 578-592; DOI:10.1098/rspb.1953.0009
- Gao Q & Horvath TL. (2007) Neurobiology of Feeding and Energy Expenditure. Annu. Rev. Neurosci. 30:367–98. doi: 10.1146/annurev.neuro.30.051606.094324
- Coleman DL. (1973) Effects of parabiosis of obese with diabetes and normal mice. Diabetologia. 9(4):294-8.
- Frederich, R. C., Löllmann, B., Hamann, A., Napolitano-Rosen, A., Kahn, B. B., Lowell, B. B., & Flier, J. S. (1995). Expression of ob mRNA and its encoded protein in rodents. Impact of nutrition and obesity. Journal of Clinical Investigation, 96(3), 1658–1663.
- Morton GJ, Meek TH, Schwartz MW. (2014) Neurobiology of food intake in health and in disease. 2014 Nature Reviews Neuroscience. 15:367-78
- Gibbs, J., Young, R. C. & Smith, G. P. (1973) Cholecystokinin decreases food intake in rats. J. Comp. Physiol. Psychol. 84, 488–495. Doi: 10.1002/j.1550-8528.1997.tb00305.x
- Gibbs J, Young RC, & Smith GP. (1973) Cholecystokinin elicits Satiety in Rats with Open Gastric Fistulas. Nature 245, 323–325. doi:10.1038/245323a0
- Turton MD, O’Shea D, Gunn I, Beak SA, Edwards CMB, Meeran K, Choi SJ, Taylor GM, Heath MM, Lambert PD, Wilding JPH, Smith DM, Ghatei MA, Herbert J, & Bloom SR. (1996) A role for glucagon-like peptide-1 in the central regulation of feeding. Nature 379, 69–72.
- Woods SC, Lotter EC, McKay LD, Porte D Jr. (1979) Chronic intracerebroventricular infusion of insulin reduces food intake and body weight of baboons. Nature. 282(5738):503-5.
- Bagdade, J. D., Bierman, E. L., & Porte, D. (1967). The Significance of Basal Insulin Levels in the Evaluation of the Insulin Response to Glucose in Diabetic and Nondiabetic Subjects. Journal of Clinical Investigation , 46 (10), 1549–1557.
- Asakawa A, Inui A, Kaga T, Yuzuriha H, Nagata T, Ueno N, Makino S, Fujimiya M, Niijima A, Fujino MA, Kasuga M. (2001) Ghrelin is an appetite-stimulatory signal from stomach with structural resemblance to motilin. Gastroenterology. 120(2):337-45.
- Aponte, Y., Atasoy, D. & Sternson, S. M. (2011) AGRP neurons are sufficient to orchestrate feeding behavior rapidly and without training. Nature Neurosci. 14, 351–355.
- Krashes, M. J. et al. (2011) Rapid, reversible activation of AgRP neurons drives feeding behavior in mice. J. Clin. Invest. 121, 1424–1428.
- Kalivas P.W., and Volkow N.D. (2005) The neural basis of addiction: a pathology of motivation and choice. Am. J. Psychiatry; 162: pp. 1403-1413
- Volkow, N. D., Wang, G.-J., & Baler, R. D. (2011). Reward, dopamine and the control of food intake: implications for obesity. Trends in Cognitive Sciences, 15(1), 37–46.
- Hommel JD, Trinko R, Sears RM, Georgescu D, Liu ZW, Gao XB, Thurmon, JJ, Marinelli M, & DiLeone RJ. (2006) Leptin Receptor Signaling in Midbrain Dopamine Neurons Regulates Feeding. Cell Volume 51, Issue 6, p801–810.
- Egecioglu E, Jerlhag E, Salomé N, Skibicka KP, Haage D, Bohlooly-Y M, Andersson D, Bjursell M, Perrissoud D, Engel JA, Dickson SL. (2010) Ghrelin increases intake of rewarding food in rodents. Addict Biol. 15(3):304-11. doi: 10.1111/j.1369-1600.2010.00216.x.
- Anand BK, Brobeck JR. (1951) Localization of a “feeding center” in the hypothalamus of the rat. Proc Soc Exp Biol Med. 77(2):323-4.
- Teitelbaum P, Epstein AN. (1962) The lateral hypothalamic syndrome: recovery of feeding and drinking after lateral hypothalamic lesions. 69():74-90.
- Sakurai T., Amemiya A., Ishii M., Matsuzaki I., Chemelli R.M., Tanaka H., et al. (1998) Orexins and orexin receptors: a family of hypothalamic neuropeptides and G protein-coupled receptors that regulate feeding behavior. Cell92: pp. 573-585
- Cason, A. M., Smith, R. J., Tahsili-Fahadan, P., Moorman, D. E., Sartor, G. C., & Aston-Jones, G. (2010). Role of orexin/hypocretin in reward-seeking and addiction: implications for obesity. Physiology & Behavior, 100(5), 419–428.
- Aston-Jones G., Smith R.J., Moorman D.E., and Richardson K.A.: Role of lateral hypothalamic orexin neurons in reward processing and addiction. Neuropharmacology 2009; 56: pp. 112-121
- Zheng H., Patterson L.M., and Berthoud H.-R. (2007) Orexin signaling in the ventral tegmental area is required for high-fat appetite induced by opioid stimulation of the nucleus accumbens. J Neurosc; 27: pp. 11075-11082
- Jennings, J. H., Ung, R. L., Resendez, S. L., Stamatakis, A. M., Taylor, J. G., Huang, J., … Stuber, G. D. (2015). Visualizing hypothalamic network dynamics for appetitive and consummatory behaviors. Cell , 160 (3), 516–527. http://doi.org/10.1016/j.cell.2014.12.026
- Opland D, Sutton A, Woodworth H, Brown J, Bugescu R, Garcia A, Christensen L, Rhodes C, Myers M Jr, Leinninger G. (2013) Loss of neurotensin receptor-1 disrupts the control of the mesolimbic dopamine system by leptin and promotes hedonic feeding and obesity. Mol Metab. 2(4):423-34.
- Murray S, Tulloch A, Gold, MS, and Avena, NM. (2014) Hormonal and neural mechanisms of food reward, eating behaviour and obesity. Nat. Rev. Endocrinol. 10, 540–552.
- Leinninger GM. Lateral thinking about leptin: a review of leptin action via the lateral hypothalamus. Physiol Behav. 2011 Sep 26;104(4):572-81. doi: 10.1016/j.physbeh.2011.04.060. Epub 2011 May 3.
- Leinninger GM, Jo YH, Leshan RL, Louis GW, Yang H, Barrera JG, Wilson H, Opland DM, Faouzi MA, Gong Y, Jones JC, Rhodes CJ, Chua S Jr, Diano S, Horvath TL, Seeley RJ, Becker JB, Münzberg H, Myers MG Jr. (2009) Leptin acts via leptin receptor-expressing lateral hypothalamic neurons to modulate the mesolimbic dopamine system and suppress feeding. Cell Metab. 10(2):89-98. doi: 10.1016/j.cmet.2009.06.011.
- Hales CM, Caroll MD, Fryar CD, & Ogden CL. (2017) Prevalence of Obesity Among Adults and Youth: United States, 2015–2016. U.S. DEPARTMENT OF HEALTH AND HUMAN SERVICES: Centers for Disease Control and Prevention: National Center for Health Statistics. NCHS Data Brief No. 288.
- Davis C, Curtis C, Levitan RD, Carter JC, Kaplan AS, Kennedy JL. (2011) Evidence that ‘food addiction’ is a valid phenotype of obesity. Appetite. 57(3):711-7. doi: 10.1016/j.appet.2011.08.017.
- Hone-Blanchet A., Fecteau S. (2014) Overlap of food addiction and substance use disorders definitions: Analysis of animal and human studies. Neuropharmacology. 85:81–90. doi: 10.1016/j.neuropharm.2014.05.019.
- Matheny M, Shapiro A, Tümer N, Scarpace PJ. (2011) Region-specific diet-induced and leptin-induced cellular leptin resistance includes the ventral tegmental area in rats. Neuropharmacology.
- Warren S. (1932) The immediate causes of death in cancer. The American Journal of the Medical Sciences. 184(5):610-615.
- Inui A (1999) Cancer anorexia-cachexia syndrome: are neuropeptides the key? Cancer Res. 59(18):4493-501.
- Valles A, Martı` O, Garcia A, Armario A. 2000. Single exposure to stressors causes long-lasting, stress-dependent reduction of food intake in rats. Am J Physiol Regul Integr Comp Physiol 279: R1138–R1144.
- Han S, Soleiman MT, Soden ME, Zweifel LS, Palmiter RD. Elucidating an Affective Pain Circuit that Creates a Threat Memory. Cell. 2015;162:363–374.
- Carter ME, Soden ME, Zweifel LS, Palmiter RD. Genetic identification of a neural circuit that suppresses appetite. Nature. 2013;503:111–114.
- Campos, C.A., Bowen, A.J., Han, S., Wisse, B.E., Palmiter, R.D., and Schwartz, M.W. Cancer-induced anorexia and malaise are mediated by CGRP neurons in the parabrachial nucleus. Nat. Neurosci. 2017; 20: 934–94 2
- Jackson VM, Breen DM, Fortin JP, Liou A, Kuzmiski JB, Loomis AK, et al. Latest approaches for the treatment of obesity. Expert Opin Drug Discov (2015)
- Palmiter, RD. (2017) Neural Circuits That Suppress Appetite: Targets for Treating Obesity. Obesity. 25(8): 1299–1301
- Herrera MF, Toouli j Kulseng B, et al. Vagal nerve block for improvements in glycemic control in obese patients with type 2 diabetes mellitus: three-year results of the VBLOC DM2 study. J Diabetes Obes 2017;4:1-6.
- Dupré DA, Tomycz N, OH M, Whiting, D. Deep brain stimulation for obesity: past, present, and future targets. Neurosurgical Focus 2015;38(6):E7.
- Whiting DM, Tomycz ND, Bailes J, de Jonge L, Lecoultre V, Wilent B, et al: Lateral hypothalamic area deep brain stimulation for refractory obesity: a pilot study with preliminary data on safety, body weight, and energy metabolism. J Neurosurg 119:56–63, 2013.