Introduction
You’ve just woken up from a deep sleep. As you slowly regain your senses, you find you have a renewed sense of vigor. The birds are chirping outside, and the sun’s rays neatly drape themselves on your wall. Your body has recharged itself, ready to take on the new day. Many animals engage in a similar form of recharging, but rather over a course of multiple weeks and months in a process called hibernation. Hibernation is key to ensuring that many animals survive harsh winters, and the captain helping the animal’s body weather this storm is the hypothalamus.
The hypothalamus is an area of the brain crucial for regulating metabolism, sleep, temperature, and other vital bodily processes. When an animal enters hibernation, the hypothalamus suppresses drives to eat, drink, and regulate body temperature, decreasing core temperature and conserving the body’s energy for long periods of time, called torpor [1]. As researchers gain insights into the mechanisms of hibernation and uncover physiological mechanisms of rebalancing energy use, their findings help us not only better understand this curious animal behavior, but also develop opportunities for treatment of human diseases.
Minimizing Energy Loss Prior to Hibernation
If you’ve ever woken up in the middle of the night with the insatiable need to have a midnight snack, you know that hunger sometimes overrides signals for sleep. The mere act of sleeping itself does not override neurological signals for hunger and thirst, which poses a problem for hibernating animals [2]. Because hibernation occurs for longer periods of time, hibernating species have evolved a variety of mechanisms to suppress food and water-seeking behaviors during hibernation.
One way in which this occurs is through suppression of ghrelin, a hormone involved in hunger that is also linked to wakefulness and activity [3]. Ghrelin stimulates feeding behaviors through activation of AMP-activated protein kinase (AMPK), which regulates food consumption [4]. AMPK is expressed in the arcuate nucleus of the hypothalamus. This, in turn, deactivates an important enzyme in fatty acid (FA) synthesis, a metabolic process that synthesizes lipid molecules from nutrients [3]. This suppression of FA synthesis decreases energy storage, indirectly encouraging energy use. The suppression of ghrelin prior to hibernation inhibits feeding behaviors by preventing AMPK from deactivating FA synthesis, allowing hibernating animals to store energy as lipids for long-term storage [3]. Additionally, ghrelin activates dopaminergic neurons in brain regions that regulate impulsive behavior, such as the ventral tegmental area, which motivate animals to get up, seek food, and consume energy in the process [5]. Therefore, the suppression of the effects of ghrelin further hinders feeding behaviors and preserves energy by suppressing activity of these dopaminergic neurons.
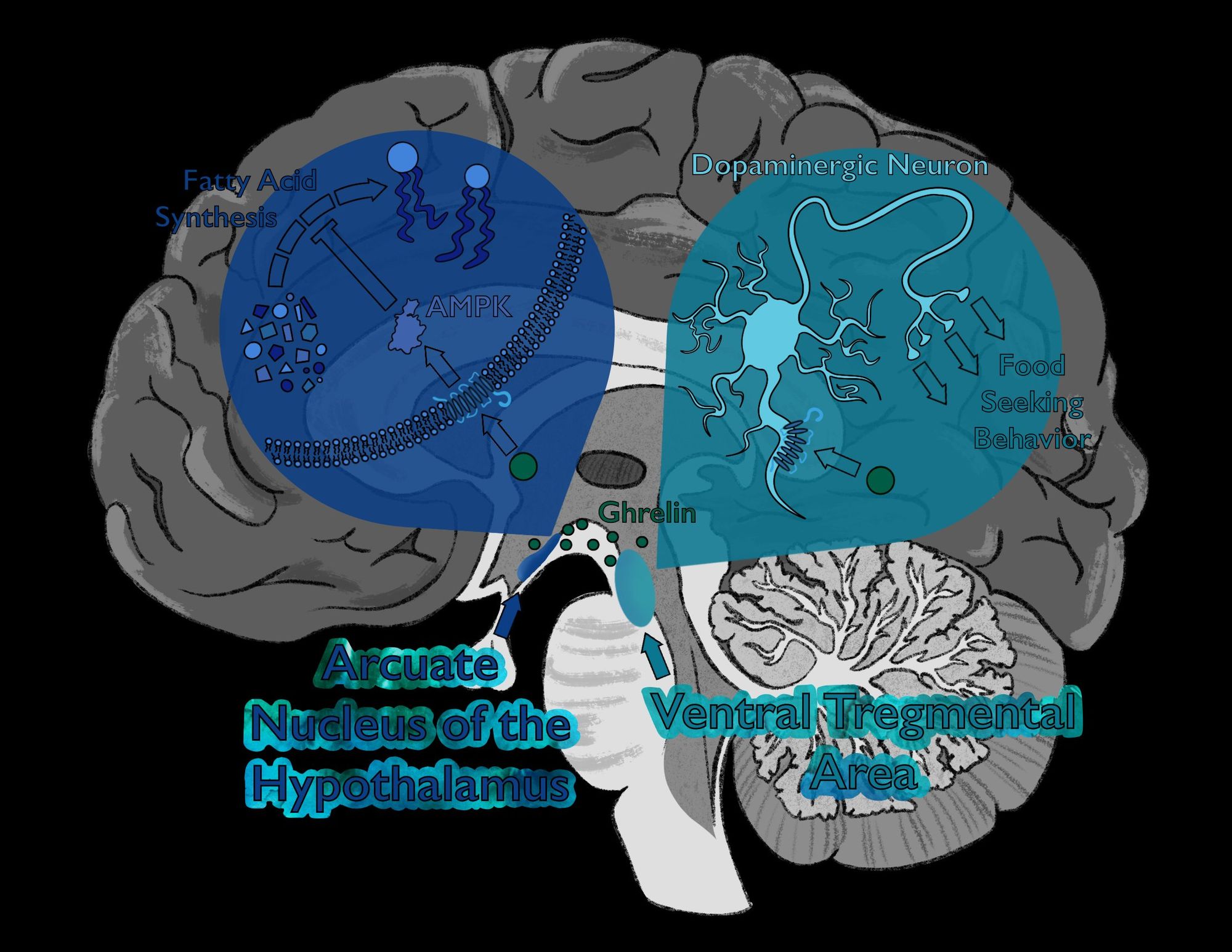
Although processes motivating food consumption are inhibited during hibernation, the amount of circulating ghrelin does not change much. In fact, even if injected with ghrelin, hibernating animals in the winter exhibit significantly lower food intake than if injected in the spring or summer when they are not hibernating [3]. Before hibernation, FA synthesis stops due to deactivation of acetyl-CoA carboxylase (ACC), an enzyme required to synthesize FAs. Thus, during hibernation, ghrelin is unable to break down FAs since they are not being produced, and with no more energy being stored in FAs, ghrelin cannot redirect this energy to be put to use [3].
Spending Energy Efficiently During Hibernation
Suppressing ghrelin prevents excessive energy loss during hibernation. However, despite this suppression, simply staying alive requires significant energy expenditure, and animals must find a way to carefully use their diminishing stores of energy throughout their hibernation. Hibernating animals have thus evolved a number of energy-preservation mechanisms, including torpor, a bout of energy conservation in which body temperature and metabolic rate decrease until only functions vital for survival are executed [1]. This keeps hibernating animals alive and well.
Many hibernating animals are heterotherms, meaning when they engage in torpor, they are able to vary between self-regulating their body temperature and equilibrating with the environment [6]. Normally, when an animal feels cold, it engages in heat-seeking behaviors such as shivering and vasoconstriction to prevent heat loss. In hibernators, however, cold sensitivity is decreased due to the decreased sensitivity of neurons expressing an ion channel called TRPM8 [7]. Researchers at Yale University performed an experiment using hibernating animals, specifically squirrels and hamsters, with non-hibernating animals that served as controls. They cloned the TRPM8 ion channel of each animal in separate frog embryos, then placed them within different temperature treatments. Frog embryos with TRPM8 ion channels from hibernating animals exhibited significantly lower sensitivity for colder temperatures when compared to their non-hibernating counterparts. More specifically, as the frog embryos were cooled from 30 to 20 degrees Celsius, the activity of TRPM8 from non-hibernating organisms increased dramatically, while the activity of TRPM8 from hibernating organisms increased only slightly. When cooled from 20 to 10 degrees, TRPM8 from hibernating animals also showed little change in activity. Additional experiments found that no external factors, but rather changes in a transmembrane residue within the TRPM8 channel itself, are responsible for this difference [7]. While the structure of TRPM8 in hibernating animals allows them to keep their body cool during hibernation, the temperature of their brains must be regulated more closely.
The brain modulates its own temperature via a protein called UCP1. Neurons expressing UCP1 are involved in generating heat within regions of the brain [8]. UCP1 is localized in the mitochondria, where it may also play a role in regulating reactive oxygen species, harmful byproducts of metabolism that accumulate over time. This ensures the brain is not damaged due to low oxygen, preserving the energy gained from metabolism [8]. This process provides much of the “rejuvenating” effect of hibernation, which is being studied for its potential therapeutic use.
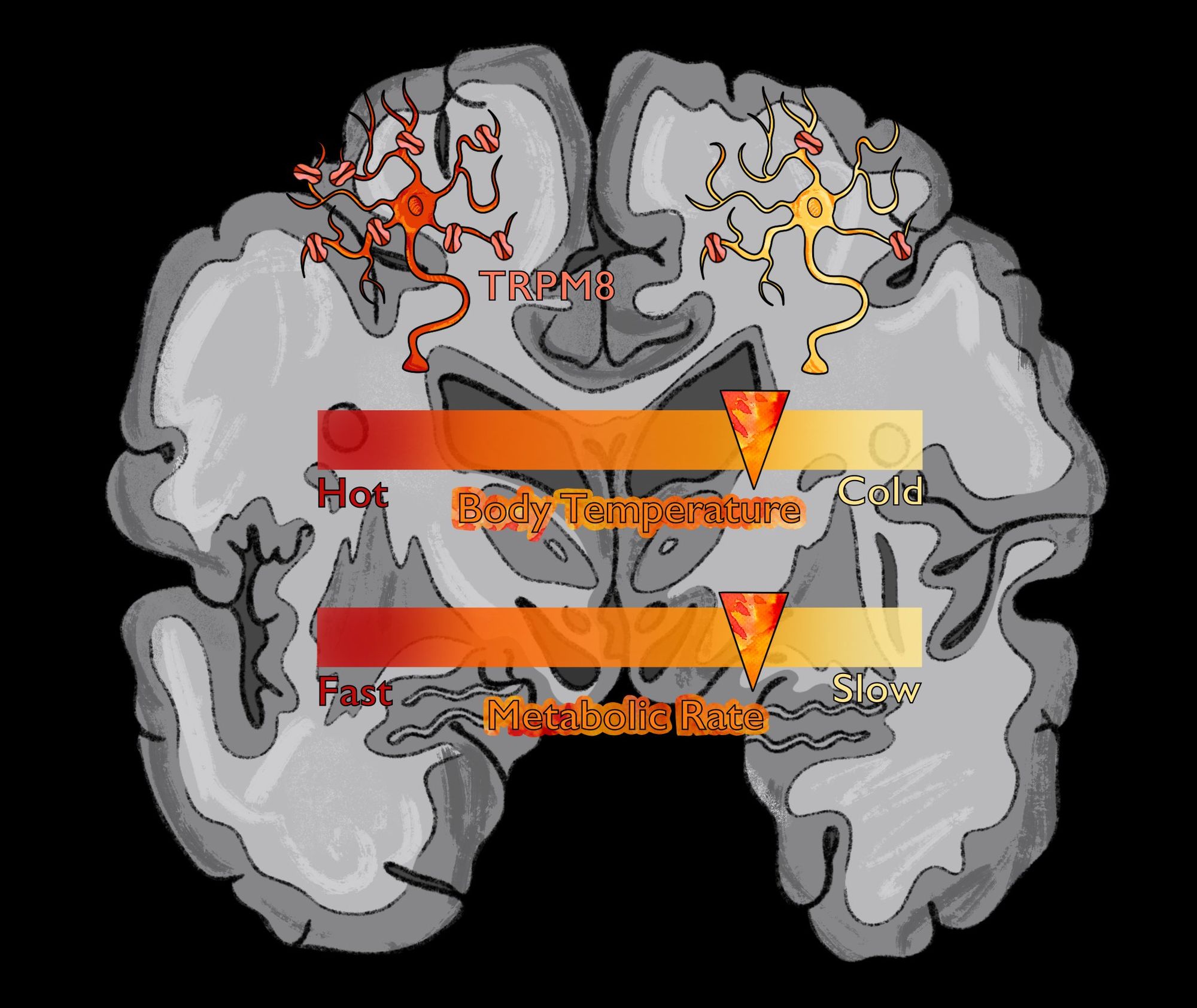
Hibernation and Humans
Animals experience many benefits from hibernation. One group of researchers identified a group of neurons within the hypothalamus that express the peptide QRFP, known as Q-neurons. Excitation of these neurons induced decreased skin temperature, locomotor activity, and low metabolism - essentially a hibernation-like state similar to torpor within rats, a non-hibernating species [9]. Some scientists now speculate whether it is possible to identify and activate this pathway within humans. While humans do not hibernate naturally, inducing hibernation-like physiological states, such as the activation of a Q-neuron analogue, may be useful forms of treatment for various diseases [3]. Low temperatures during these hibernation-like states have been found to suppress inflammatory pathways, preventing brain damage during strokes [10]. Researchers at the University of Leipzig found that a torpor-state may be helpful in combating Alzheimer’s disease (AD) [11]. One hallmark of AD is the phosphorylation of tau, a process that leads to development of neurofibrillary tangles implicated in the disease. This process is normally irreversible, leading to neuronal death. However, the aggregation of tau proteins is necessary as the body enters a low energy state, but is subsequently reversed when the animal wakes. The researchers showed that animals that were aroused after being in a state of hibernation exhibited reversible tau phosphorylation. Thus, the researchers proposed that hibernation could help reverse this process in humans as well, presenting a novel treatment for Alzheimer’s disease [11]. In order to fully determine whether this might be possible, much more research is needed on the mechanisms behind both reversible tau aggregation and its relationship to Alzheimer’s Disease.
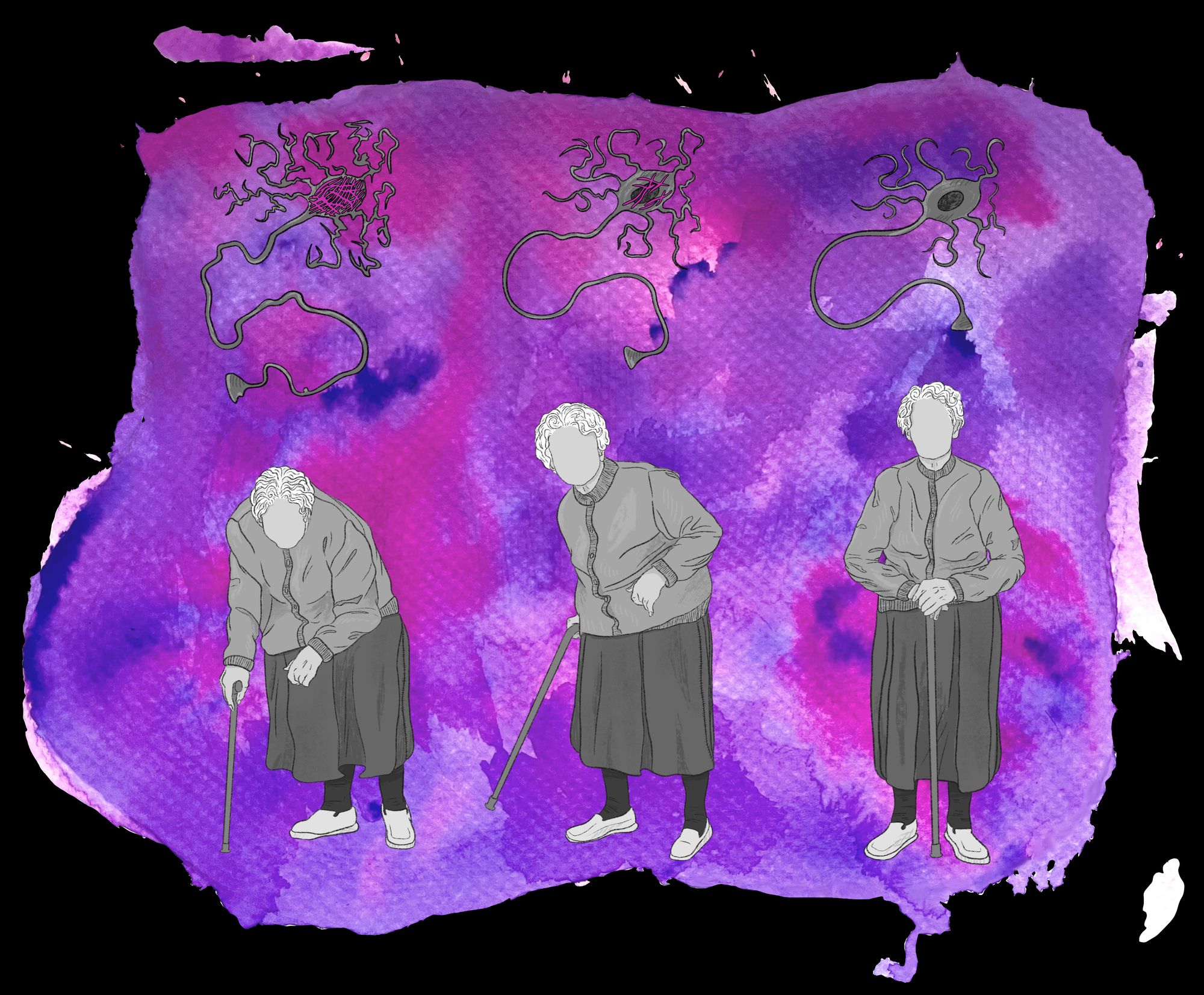
When we think of a little squirrel, laid cozily in its burrow, awaiting the sunshine of spring, we often seek to emulate the seemingly endless inertia, completely unphased by the world around us. However, within the squirrel, there is a careful balance being constructed, minimizing energy exchange and temperature variation. It is this balance, not the inertia, that allows the squirrel to emerge rejuvenated. The nervous system allows the squirrel to carefully walk this line and, in turn, emerge better from it.
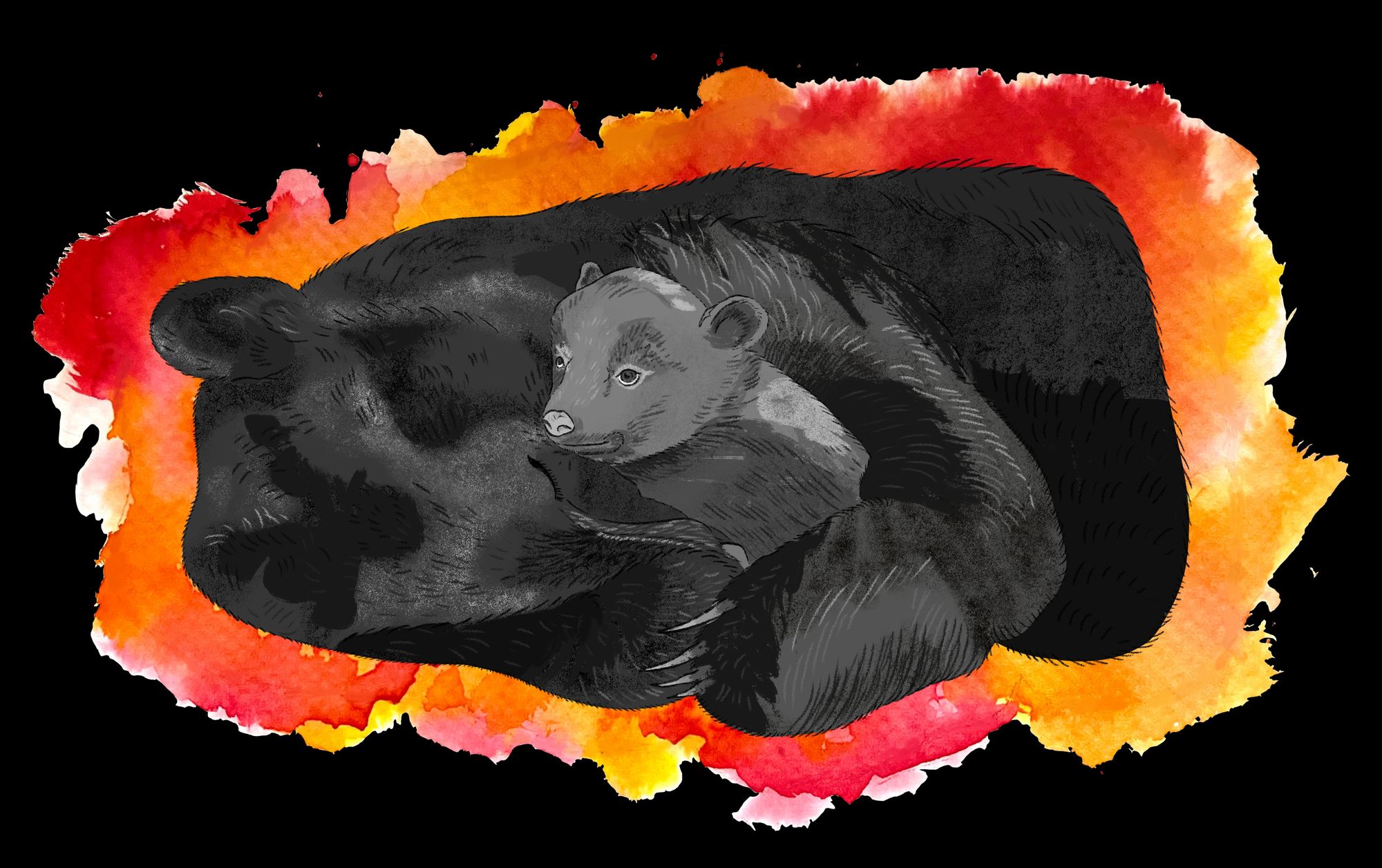
References
- Junkins, M. S., Bagriantsev, S. N., & Gracheva, E. O. (2022). Towards understanding the neural origins of hibernation. Journal of Experimental Biology, 225(1):jeb229542. https://doi.org/10.1242/jeb.229542
- Goldstein, N., Levine, B. J., Loy, K. A., Duke, W. L., Meyerson, O. S., Jamnik, A. A., & Carter, M. E. (2018). Hypothalamic Neurons that Regulate Feeding Can Influence Sleep/Wake States Based on Homeostatic Need. Current Biology: CB, 28(23), 3736–3747.e3. https://doi.org/10.1016/j.cub.2018.09.055
- Healy, J. E., Bateman, J. L., Ostrom, C. E., & Florant, G. L. (2011). Peripheral ghrelin stimulates feeding behavior and positive energy balance in a sciurid hibernator. Hormones and Behavior, 59(4), 512-519. https://doi.org/10.1016/j.yhbeh.2011.01.013
- Oh, T. S., Cho, H., Cho, J. H., Yu, S. W., & Kim, E. K. (2016). Hypothalamic AMPK-induced autophagy increases food intake by regulating NPY and POMC expression. Autophagy, 12(11), 2009–2025. https://doi.org/10.1080/15548627.2016.1215382
- Anderberg, R. H., Hansson, C., Fenander, M., Richard, J. E., Dickson, S. L., Nissbrandt, H., Bergquist, F., & Skibicka, K. P. (2015). Neuropsychopharmacology, 41, 1199-1209. https://doi.org/10.1038/npp.2015.297
- Geiser, F. (2013). Hibernation. Current Biology: CB, 23(5), R188-R193. https://doi.org/10.1016/j.cub.2013.01.062
- Matos-Cruz, V., Schneider, E. R., Mastrotto, M., Merriman, D. K., Bagriantsev, S. N., & Gracheva, E. O. (2017). Molecular prerequisites for diminished cold sensitivity in ground squirrels and hamsters. Cell Reports, 21(12), 3329-3337. https://doi.org/10.1016/j.celrep.2017.11.083
- Laursen, W. J., Mastrotto, M., Pesta, D., & Gracheva, E. O. (2015). Neuronal UCP1 expression suggests a mechanism for local thermogenesis during hibernation. Proceedings of the National Academy of Sciences of the United States of America (PNAS), 112(5), 1607-1612. https://doi.org/10.1073/pnas.1421419112
- Takahashi, T. M., Sunagawa, G. A., Soya, S., Abe, M., Sakurai, K., Ishikawa, K., Yanagisawa, M., Hama, H., Hasegawa, E., Miyawaki, A., Sakimura, K., Takahashi, M., & Sakurai, T. (2020). A discrete neuronal circuit induces a hibernation-like state in rodents. Nature, 583, 109-114. https://doi.org/10.1038/s41586-020-2163-6
- Liesz, A., Zhou, W., Mracskó, É., Karcher, S., Bauer, H., Schwarting, S., Sun, L., Bruder, D., Stegemann, S., Cerwenka, A., Sommer, C., Dalpke, A. H., & Veltkamp, R. (2011). Inhibition of lymphocyte trafficking shields the brain against deleterious neuroinflammation after stroke. Brain, 134(3), 704-720. https://doi.org/10.1093/brain/awr008
- Arendt, T., Stieler, J., Strijkstra, A. M., Hut, R. A., Rüdiger, J., Van der Zee, E. A., Harkany, T., Holzer, M., & Härtig, W. (2003). Reversible Paired Helical Filament-Like Phosphorylation of Tau Is an Adaptive Process Associated with Neuronal Plasticity in Hibernating Animals. The Journal of Neuroscience, 23(18), 6972-6981. https://doi.org/10.1523/JNEUROSCI.23-18-06972.2003