Superman: Man or Myth?
On an unforgettable spring day in 1995, esteemed Superman actor Christopher Reeve redefined the meaning of his role as the “man of steel.” Once recognized as a legendary symbol of superhuman strength, Reeve endured a drastic life change when a fall during an equestrian competition that morning left him paralyzed from the neck down. The accident broke the first and second vertebrae in his neck, leaving him with a severe spinal cord injury (SCI). Hemorrhaging at the contact site damaged the nerve fibers responsible for important signals that connect the brain to the body. From that point onward, the superstar found himself irrevocably confined to a wheelchair, reliant on a ventilator for his every breath.
Christopher Reeve represents just one of many individuals with SCI. More than 300,000 people in the United States are affected by these injuries, most of which are induced by traumatic accidents [1]. Severe cases, which comprise about a third of all SCI in the U.S., lead to permanent paralysis. Over 97% of individuals affected by complete SCI paralysis never regain the ability to perform tasks as seemingly basic as reaching for and grasping objects, actions we typically take for granted in our daily lives [2]. These alarming numbers underscore the critical need for continued research and advancements in SCI treatment and care.
Despite these sobering statistics, scientists have struggled to find a cure due to the complexity of the nervous system. As a result, the life expectancy for individuals with SCI has seen limited improvement since the 1980s, and the absence of consistently effective treatments still leaves nearly a third of patients rehospitalized after the initial injury. With medical procedures and treatments growing increasingly expensive, SCI also imposes a heavy financial burden upon patients [3, 4]. For many affected individuals, the hope of overturning their paralysis remains but a distant dream.
Considering his complete loss of motor and sensory function below the injury site, Reeve’s doctors had little hope for his recovery. So severe was his SCI, and so uncertain were the effects of available treatment, that both he and his immediate family considered ending his life. Still, Reeve pursued a solution. Intensive physical therapy combined with functional electrical stimulation (FES), a common method of treatment that strengthens paralyzed muscle groups via the pedaling of a computer-assisted electric bicycle, yielded impressive results. After a rigorous five-year treatment period, Reeve regained his sense of touch and control of minor muscle movement [4].
Unfortunately, FES treatment is not always effective. Studies measuring motor function after FES treatment show inconsistent improvements among different patients. FES also lacks the ability to target certain anatomical sites, which prevents specific damaged nerve fibers from being reactivated [5]. Hence, there remains a pressing need to find additional treatments for SCI.
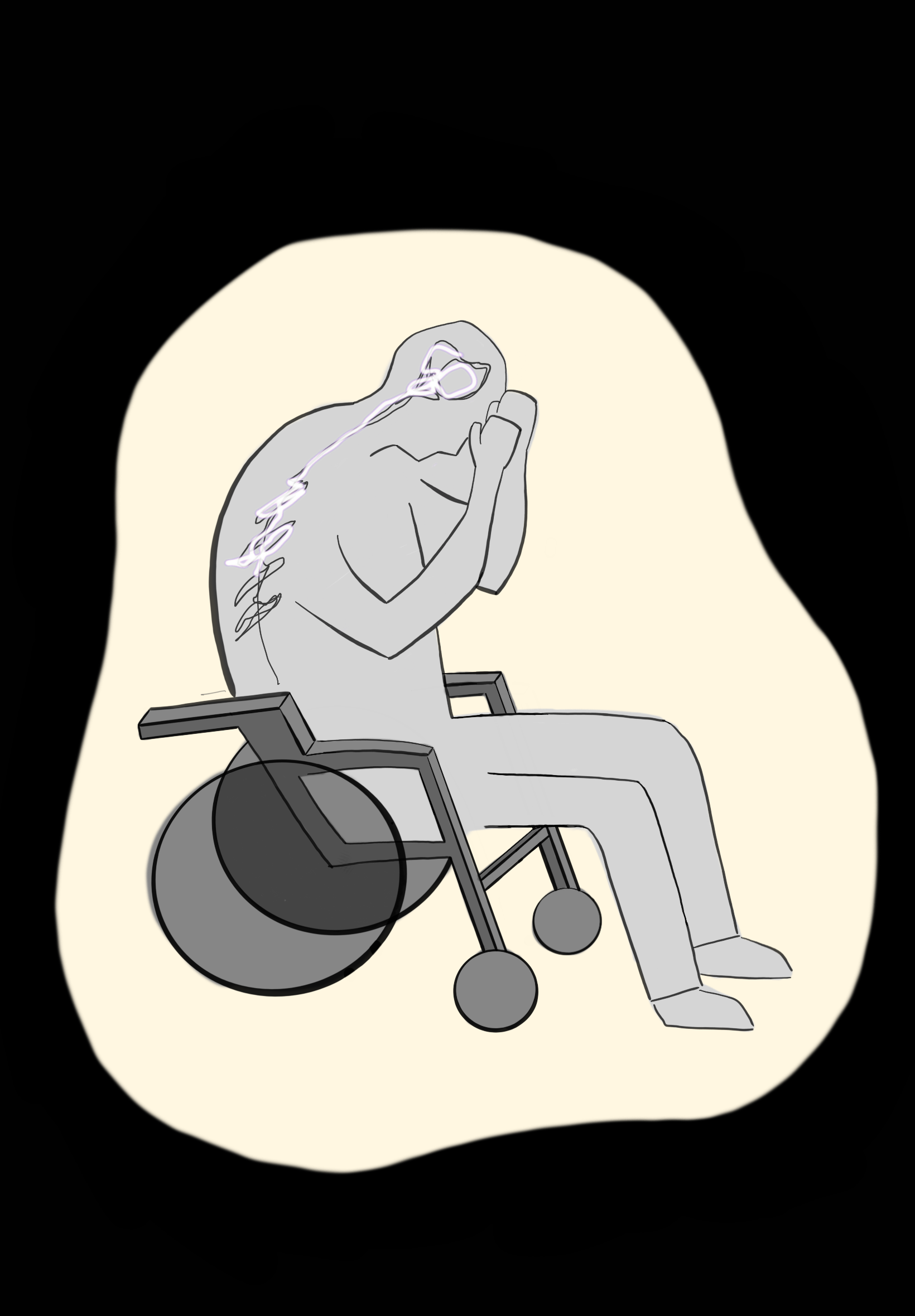
A Popular Perception of Regenerative Medicine: Stem Cell Therapy
While activity-based restorative therapy such as FES remained integral to SCI recovery, scientists of the late 20th century began to explore the prospects of regenerative medicine, an approach aimed at replacing or regrowing damaged tissues to restore function. This exploration was driven by the recognition that the body’s natural healing mechanisms alone could not fully repair the effects of SCI [1]. Despite the central nervous system’s renowned plasticity that enables spontaneous recovery from various injuries, it actually possesses little ability to replace neurons damaged by SCI with new cells. On the outside of the injury, glial scars form as visible evidence of the body’s attempt to contain and isolate immediate damage, but inhibitory molecules within these scars prevent spinal tissue from restoring long-term function [1]. Faced with these challenges, scientists sought to leverage other biochemical mechanisms within the body as potential therapeutic avenues.
Stem cell research, widely recognized as the epitome of regenerative medicine, has emerged as an especially groundbreaking field. By harnessing the remarkable potential of stem cells to mature into specialized cells with distinct functions, scientists have opened up new possibilities for treating a wide range of medical conditions. Researchers theorize that transplanting stem cells into individuals with SCI will facilitate the development of healthy tissues, effectively replacing those that were harmed by the injury [6].
Today, stem cells are still thoroughly researched as an avenue for SCI repair. Extensive research in rats shows promising findings related to the use of stem cell therapy in SCI. For instance, treatment with one type of stem cell rebuilds myelin, a protective fatty layer surrounding axons that is damaged by SCI. This myelin restoration is essential for insulating neurons and facilitating rapid communication between them [7]. Additionally, stem cells have the potential to replace damaged astrocytes in order to provide necessary support and nutrition, or even restore neuronal circuitry by replacing damaged neurons [6]. As the progress in stem cell research unfolds, it brings forth new possibilities for SCI treatment, instilling a renewed sense of optimism for those affected by these debilitating injuries.
However, stem cells have critical shortcomings. While we can generally culture and transplant stem cells with ease, we have yet to learn how to differentiate stem cells into their appropriate neuronal phenotypes so that they function effectively in the body. Furthermore, we currently do not have the technology to guarantee the long-term survival of all stem cell types in the body [8]. Not all stem cells are biocompatible, and those that do possess biocompatible delivery mechanisms are not necessarily permeable to important proteins. Even if the issue of biocompatibility is resolved, stem cells face the problem of de-differentiation, wherein they revert to an earlier cell stage and become less specialized [9]. As researchers continue to unravel the complexities of stem cell therapy for SCI, addressing these challenges will pave the way for advancements in the field.
In addition to the challenges related to stem cell differentiation and long-term survivability, the identification of a consistent target area for treatment poses a difficulty due to the variability in the size and location of an SCI in different patients [10]. For larger injuries, providing mechanical support becomes paramount to facilitate healing, and it is critical to use a delivery material that is compatible with the microenvironment of the lesion site. However, it is important to note that the realm of regenerative medicine extends well beyond stem cells. Just as reengineered stem cells can deliver cellular therapy to the body, current research indicates that modifying other naturally occurring structures within the body can provide novel avenues for transporting therapeutic agents to treat SCI [11]. This endeavor addresses the intricate challenges of stem cell differentiation and biocompatibility, presenting the potential to revolutionize SCI treatment and the field of regenerative medicine.
Different Perspectives in Regeneration: Supramolecular Polymers
As a mother would feed medicine to an uncooperative child by hiding it in a spoonful of delicious cake, bioengineers design artificial structures that can sneak past the immune system in order to perform functions in the body. Many researchers have experimented with small structures called “supramolecular polymers” to act as the metaphorical cake for SCI treatment. These polymers are constructed from proteins and organic molecules such as DNA [11]. Due to their biocompatibility with the body, these agents can deliver therapeutic materials to the site of injury without being flagged as a threat [12].
Supramolecular polymers also possess the power to self-assemble into their polymeric state from monomeric parts [12]. These complexes can dynamically transition between the two states because of the weak bonding forces between their components. The reversible nature of their assembly empowers supramolecular polymers to transiently create distinct chemical architectures that lead to dynamic interactions with surrounding molecules. Current research shows that mediating these changes can increase their lifetime in the body, allowing for the polymers to fulfill their therapeutic potential more effectively before undergoing degradation [12].
Small molecules called peptide amphiphiles (PAs) are frequently used to build these supramolecular systems [13]. Composed of a protein sequence linked to a tail of hydrophobic atoms, PAs can be designed to form twisted “beta sheet” polypeptide chains by rearranging the protein’s amino acids. The resulting beta sheets hold a large capacity for carrying biological signals on their surface. Analogous to how a lock fits a key, each of these signals can bind to a corresponding signal or receptor molecule in the body. This binding process activates the pathway associated with each signal-receptor molecule pair [13]. Given the dynamic nature of supramolecular polymer assembly, the temporal structure of supramolecular polymers plays a pivotal role in determining the interactions between signals and receptors [14].
Importantly, PA molecules possess properties that make them especially advantageous for SCI treatment. Research by the Stupp Lab at Northwestern University revealed that PA molecules could self-assemble into three-dimensional structures that are optimal for retaining large amounts of water [15]. The elasticity, fibrosity, and exceptional water absorbance of PAs enable them to closely mirror the features of the extracellular matrix (ECM), a non-cellular component present in all tissue. Imitating the role of the ECM, this synthetic mimicry provides structural support and crucial biochemical cues that regulate the fate of surrounding cells [16]. When integrated into the site of an SCI, these properties enable structural repair and facilitate the chemical pathways crucial for the recovery process.
In recent years, the Stupp Lab has investigated the use of PAs to counteract these obstructive effects on axonal regeneration. Their injectable therapy, “dancing molecules,” suggests the use of ECM-mimicking supramolecular polymers for delivering signals into the body that treat SCI [17]. By modifying the protein sequences within PAs, scientists discovered that specific designs increased the mobility of PAs, resulting in frequent collisions and enhanced activation of signaling pathways. This dynamic interaction between molecules could be visualized as a graceful “dance”. To evaluate the effectiveness of the “dancing molecules” therapy, mice with severe paralysis were treated with these modified PAs. Only four weeks later, the rodents demonstrated significant improvement in their ability to walk [17].
The Molecular Tango that Restores Mobility: Dancing Molecules
With axon regeneration and the regrowth of neurons being two primary measures of SCI repair, researchers must modify PAs to carry signals that promote these actions [18]. Hence, researchers in the Stupp Lab integrated two special signals into their PA design. The first was IKVAV, a signaling peptide molecule which facilitates axon regeneration and the development of neural stem cells into mature neurons. Previous research in mice demonstrated that incorporating IKVAV signals into PA supramolecular polymer therapies could restore partial motor function after SCI [18]. When administered only the IKVAV-PA treatment, mice recovered the ability to step on the dorsal side of their feet, a position which almost resembles dragging.
Results improved significantly upon the addition of a second special signal to the PA. This second molecule was fibroblast growth factor-2 (FGF-2), a peptide which helps activate pathways that stimulate cell proliferation and survival [19]. Scientists added both signals to the end of the PA molecules near the protein sequences, ultimately creating the structure of an optimized “dancing molecule” [17]. Researchers in the Stupp Lab determined that slight mutations of the dancing molecule protein sequences could lead to significant increases in the motion of the whole polymer. They hypothesized that this increased molecular motion could promote SCI repair.
The mystery behind the success of the dancing molecule structure can be explained by a simple mechanism involving electromagnetic forces between molecules [13]. The polarity of protein sequences affects the ability of supramolecular polymers to form beta sheets, which tend to coil together due to attractive hydrogen-bond interactions between their molecules. In other words, strong hydrogen bonding in the protein sequences can compress beta sheet polypeptides, similar to how a copious amount of glue firmly attaches two objects. This resulting structure hampers the free motion of molecules within the supramolecular polymer, effectively impeding its activity. Conversely, in the case of dancing molecules, a lack of hydrogen bonding offers the supramolecular polymer greater freedom of motion, akin to how the absence of glue makes two glued objects more prone to separating [13].
Researchers in the Stupp Lab found that the amino acid sequences IKVAV PA2 and FGF2 PA1 yielded not only the most motion, but also exhibited the strongest indicators of functional recovery from SCI [17]. Mice treated with this high-motion protein therapy demonstrated a robust regrowth of axons that facilitated motor function in the injured area, as well as an increase in blood vessel formation to form new tissue, reduction in glial scar density which can help enable healing, and improved cellular survival. Additionally, these animals consistently showed a significant increase in stride length and width when walking, indicating that the cellular effects of these PAs translated into functional recovery [17].
Although data thoroughly establishes the impact of supramolecular motion on functional recovery, the precise underlying mechanisms remain elusive to scientists. Rather, experimental data only suggests that a high-motion supramolecular polymer may exhibit enhanced interactions with signaling molecules and receptors in the body [17]. One theory to explain this phenomenon suggests that when the polymer is able to freely move into different conformations, its flexibility increases the likelihood that the molecule will bind to the receptors needed to initiate SCI repair pathways. Alternatively, the recovery process could be attributed to the beneficial interactions of the supramolecular polymer nanostructures with the proteins of the ECM [17]. Further research is needed to discern the exact mechanism responsible for the success of “dancing molecules”.
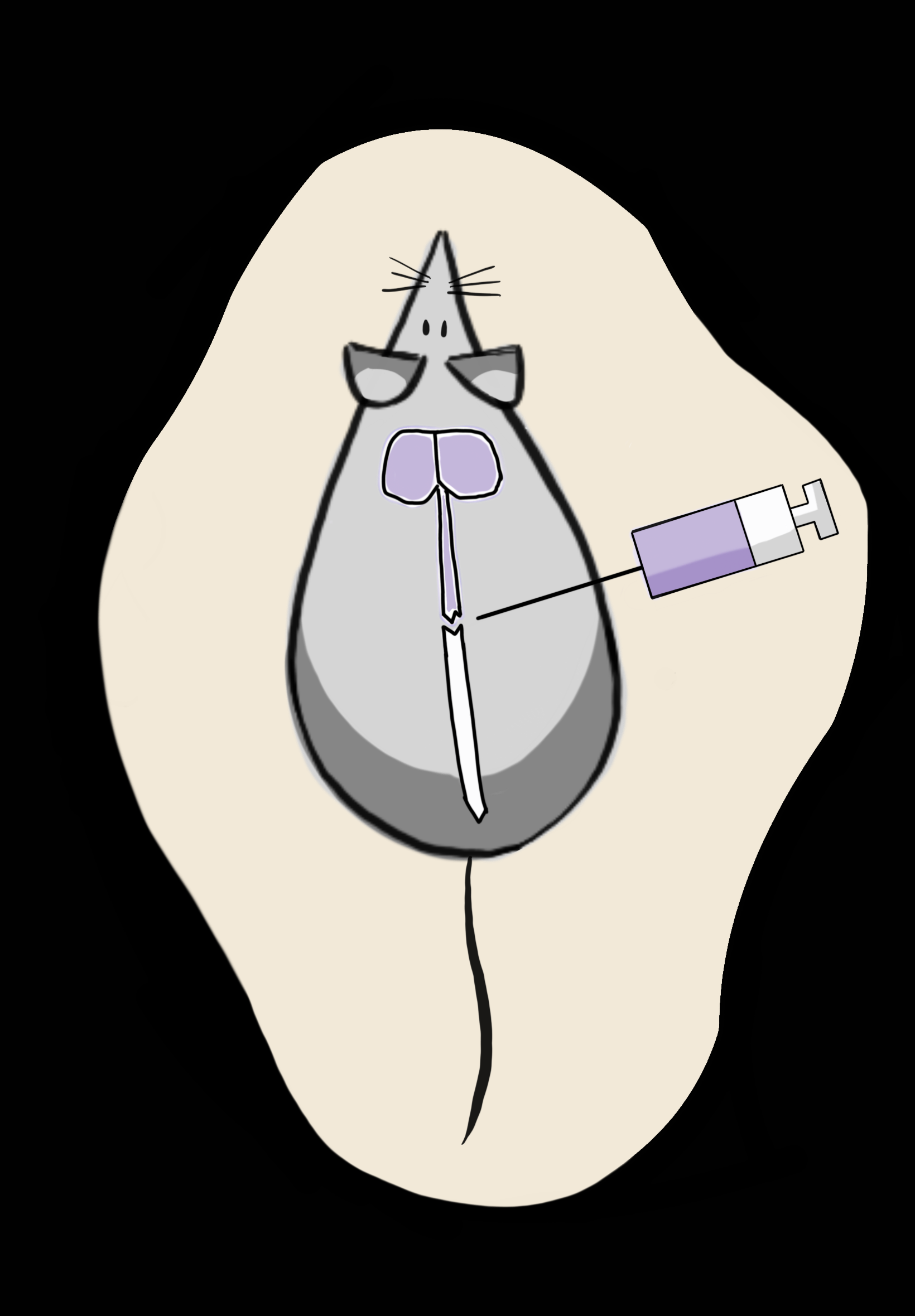
Extended Applications of Stem Cells & Supramolecular Polymers
Apart from IKVAV and FGF2, there are other molecules that can be incorporated into supramolecular polymers. One line of research shows that these polymers can also integrate and activate a protein found in the nervous system called brain-derived neurotrophic factor (BDNF) [20]. BDNF plays a role in facilitating the production and maturation of neuronal cells and controlling how effectively neurons communicate after disease. In previous studies, administering additional BDNF after an injury led to limited success due to its inability to bind with the specific receptor. By integrating BDNF into a supramolecular peptide, it can successfully bind to its receptor, thereby activating the corresponding signaling pathway. Researchers speculate that the cause of this success can be attributed to the dynamic behavior of the PA monomers, which are able to self-assemble into an optimal polymer configuration [20].
Scientists are also investigating the combination of stem cells and supramolecular polymers in an effort to create a unified mechanism which possesses the benefits of both units. Due to inherent biological differences between stem cells and the damaged microenvironment, stem cells are often inserted into the body by using a biocompatible delivery material [11]. This biocompatibility and biodegradability has been studied extensively by scientists. However, research rarely recognizes the importance of the permeability of delivery materials, which helps nutrients and signal molecules to interact with stem cells. Supramolecular polymers offer the ability to bypass the immune system’s defenses and permeate important proteins, thereby facilitating the effective delivery of therapeutic effects in the body [11].
The use of supramolecular polymers as a three-dimensional, permeable scaffold that carries neural stem cells has demonstrated measurable success in reversing paralysis in rats. Specifically, this three-dimensional matrix ensures a more uniform distribution of signal molecules from the body, stopping growth-inhibitory molecules from accumulating at injury sites. After treatment, the rats not only reestablished neural networks via the growth and maturation of stem cells but also recovered voluntary hindlimb motion [11]. These promising outcomes highlight the combined potential of supramolecular polymers and stem cells in reversing paralysis, inspiring further exploration in the field of regenerative medicine.
Scientists are also investigating the ability of supramolecular polymers to transport neural progenitor cells into the body. Neural progenitor cells are similar to stem cells, but they can only develop into cells that are part of the nervous system. Experiments revealed positive effects when supramolecular polymers incorporating IKVAV and neural progenitor cells were injected into the body [17]. Notably, the supramolecular polymer demonstrated that it could help induce the rapid maturation of progenitor cells into neurons faster than other peptide types that are more commonly used to carry stem cells. Moreover, the supramolecular polymer has shown the ability to inhibit the development of neural progenitor cells into cells that impede regenerative processes. This specific characteristic of progenitor cell maturation has been linked to the increased density of IKVAV signaling peptides on the polymer’s surface [17]. Altogether, the integration of stem cell and supramolecular polymer research has introduced valuable findings with the potential to radically transform medicine.
The Return of Superman: A Possibility of the Once Impossible
Although the combination of stem cells and supramolecular polymers is exciting, there are critical issues that must be addressed. Stem cells, even those that are derived from humans, may present complications such as the formation of abnormal stem cell colonies and tumors, which can hinder regenerative processes in SCI [18]. Furthermore, concerns regarding the ethics and safety of certain stem cell transplantation have yet to be resolved. Ultimately, the use of supramolecular polymers in medical research has not been studied extensively enough to ensure their safe application in human beings.
Stem cells and supramolecular polymers, however, aren’t the only regenerative treatments that are being researched. Ongoing research also focuses on other pharmacological drugs with potential roles in SCI recovery [17]. These drugs aim to amplify the expression of certain growth factors, regulate immune response biomolecules, and activate ion channels involved in neuronal signaling using nanoparticles. Experimental trials using animal models have reported substantial cellular and physiological recovery in response to these treatments [17].
Despite advancements in these therapies reaching the preclinical stage, an official approval by the U.S. Food and Drug Administration has yet to be acquired [17]. Reproducible positive results from human clinical trials are critical before regenerative medicine can be implemented in hospitals [21]. The anatomy of model animals like rats and mice differs fundamentally from humans, so effects observed in injured animals may not directly translate to humans with SCI.
Until sufficient research is conducted, regenerative medicine will remain in its experimental stages. However, it is worth emphasizing that stem cells and supramolecular polymers, despite being in their infancy, hold immense promise for future treatments. Just as Superman inspires us with his ability to save the day, the remarkable potential of these transformative agents ignites a beacon of hope for those affected by SCI. In the future, these extraordinary tools may hold the key to unlocking the full reversal of paralysis in humans, surpassing our current limitations and ultimately transforming lives.
References
- Bradbury, E. J., & Burnside, E. R. (2019). Moving Beyond the Glial Scar for Spinal Cord Repair. Nature Communications, 10(1). https://doi.org/10.1038/s41467-019-11707-7
- National Signal Cord Injury Statistical Center. (2022). NSCISC. University of Alabama Birmingham. https://msktc.org/sites/default/files/SCI-Facts-Figs-2022-Eng-508.pdf.
- Harvey, C., Wilson, S. E., Greene, C. G., Berkowitz, M., & Stripling, T. E. (1992). New Estimates of the Direct Costs of Traumatic Spinal Cord Injuries: Results of a Nationwide Survey. Paraplegia, 30(12), 834–850. https://doi.org/10.1038/sc.1992.160
- McDonald, J. W., Becker, D., Sadowsky, C. L., Jane, J. A., Conturo, T. E., & Schultz, L. M. (2002). Late Recovery Following Spinal Cord Injury. Journal of Neurosurgery: Spine, 97(2), 252–265. https://doi.org/10.3171/spi.2002.97.2.0252
- (C) Duffell, L. D., & Donaldson, N. de. (2020). A Comparison of FES and SCS for Neuroplastic Recovery After SCI: Historical Perspectives and Future Directions. Frontiers in Neurology, 11. https://doi.org/10.3389/fneur.2020.00607
- (O) Nandoe Tewarie, R. S., Hurtado, A., Bartels, R. H., Grotenhuis, A., & Oudega, M. (2009). Stem Cell-based Therapies for Spinal Cord Injury. The Journal of Spinal Cord Medicine, 32(2), 105–114. https://doi.org/10.1080/10790268.2009.11760761
- (3) Razavi, S., Ghasemi, N., Mardani, M., & Salehi, H. (2017). Remyelination Improvement After Neurotrophic Factors Secreting Cells Transplantation in Rat Spinal Cord Injury. Iranian Journal of Basic Medical Sciences, 20(4), 392–398. https://doi.org/10.22038/IJBMS.2017.8580
- (5) Qu, J., & Zhang, H. (2017). Roles of Mesenchymal Stem Cells in Spinal Cord Injury. Stem Cells International, 2017, 5251313. https://doi.org/10.1155/2017/5251313
- (P) Zietlow, R., Lane, E. L., Dunnett, S. B., & Rosser, A. E. (2007). Human Stem Cells for CNS Repair. Cell and Tissue Research, 331(1), 301–322. https://doi.org/10.1007/s00441-007-0488-1
- (6) Shao, A., Tu, S., Lu, J., & Zhang, J. (2019). Crosstalk Between Stem Cell and Spinal Cord Injury: Pathophysiology and Treatment Strategies. Stem Cell Research & Therapy, 10(1). https://doi.org/10.1186/s13287-019-1357-z
- (A) Yuan, T., Shao, Y., Zhou, X., Liu, Q., Zhu, Z., Zhou, B., Dong, Y., Stephanopoulos, N., Gui, S., Yan, H., & Liu, D. (2021). Highly Permeable DNA Supramolecular Hydrogel Promotes Neurogenesis and Functional Recovery After Completely Transected Spinal Cord Injury. Advanced Materials, 33(35), 2102428. https://doi.org/10.1002/adma.202102428
- (7) Aida, T., Meijer, E. W., & Stupp, S. I. (2012). Functional Supramolecular Polymers. Science, 335(6070), 813–817. https://doi.org/10.1126/science.1205962
- (D) Hendricks, M. P., Sato, K., Palmer, L. C., & Stupp, S. I. (2017). Supramolecular Assembly of Peptide Amphiphiles. Accounts of Chemical Research, 50(10), 2440–2448. https://doi.org/10.1021/acs.accounts.7b00297
- Pavlović, R. Z., Egner, S. A., Palmer, L. C., & Stupp, S. I. (2023). Supramolecular Polymers: Dynamic Assemblies of “Dancing” Monomers. Journal of Polymer Science, 61(10), 870–880. https://doi.org/10.1002/pol.20230115
- Cui, H., Webber, M. J., & Stupp, S. I. (2010). Self-Assembly of Peptide Amphiphiles: From Molecules to Nanostructures to Biomaterials. Biopolymers, 94(1), 1–18. https://doi.org/10.1002/bip.21328
- (E) Frantz, C., Stewart, K. M., & Weaver, V. M. (2010). The Extracellular Matrix at a Glance. Journal of Cell Science, 123(Pt 24), 4195–4200. https://doi.org/10.1242/jcs.023820
- (J) Álvarez, Z., Kolberg-Edelbrock, A. N., Sasselli, I. R., Ortega, J. A., Qiu, R., Syrgiannis, Z., Mirau, P. A., Chen, F., Chin, S. M., Weigand, S., Kiskinis, E., & Stupp, S. I. (2021). Bioactive Scaffolds with Enhanced Supramolecular Motion Promote Recovery from Spinal Cord Injury. Science (New York, N.Y.), 374(6569), 848–856. https://doi.org/10.1126/science.abh3602
- (K) Silva, G. A., Czeisler, C., Niece, K. L., Beniash, E., Harrington, D. A., Kessler, J. A., & Stupp, S. I. (2004). Selective Differentiation of Neural Progenitor Cells by High-epitope Density Nanofibers. Science, 303(5662), 1352–1355. https://doi.org/10.1126/science.1093783
- (L) Rubert Pérez, C. M., Álvarez, Z., Chen, F., Aytun, T., & Stupp, S. I. (2017). Mimicking the Bioactivity of Fibroblast Growth Factor-2 Using Supramolecular Nanoribbons. ACS Biomaterials Science & Engineering, 3(9), 2166–2175. https://doi-org.offcampus.lib.washington.edu/10.1021/acsbiomaterials.7b00347
- (B) Edelbrock, A. N., Àlvarez, Z., Simkin, D., Fyrner, T., Chin, S. M., Sato, K., Kiskinis, E., & Stupp, S. I. (2018). Supramolecular Nanostructure Activates Trkb Receptor Signaling of Neuronal Cells By Mimicking Brain-derived Neurotrophic Factor. Nano Letters, 18(10), 6237–6247. https://doi.org/10.1021/acs.nanolett.8b02317
- Ashammakhi, N., Kim, H.-J., Ehsanipour, A., Bierman, R. D., Kaarela, O., Xue, C., Khademhosseini, A., & Seidlits, S. K. (2019). Regenerative Therapies for Spinal Cord Injury. Tissue Engineering Part B: Reviews, 25(6), 471–491. https://doi.org/10.1089/ten.teb.2019.0182